Popular information
Popular science background:
How the optical microscope became a nanoscope (pdf)
Populärvetenskaplig information:
Hur ljusmikroskopet blev ett nanoskop (pdf)
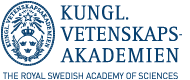
The Nobel Prize in Chemistry 2014
Eric Betzig, Stefan W. Hell and William E. Moerner are awarded the Nobel Prize in Chemistry 2014 for having bypassed a presumed scientific limitation stipulating that an optical microscope can never yield a resolution better than 0.2 micrometres. Using the fluorescence of molecules, scientists can now monitor the interplay between individual molecules inside cells; they can observe disease-related proteins aggregate and they can track cell division at the nanolevel.
How the optical microscope became a nanoscope
Red blood cells, bacteria, yeast cells and spermatozoids. When scientists in the 17th century for the first time studied living organisms under an optical microscope, a new world opened up before their eyes. This was the birth of microbiology, and ever since, the optical microscope has been one of the most important tools in the life-sciences toolbox. Other microscopy methods, such as electron microscopy, require preparatory measures that eventually kill the cell.
Glowing molecules surpassing a physical limitation
For a long time, however, optical microscopy was held back by a physical restriction as to what size of structures are possible to resolve. In 1873, the microscopist Ernst Abbe published an equation demonstrating how microscope resolution is limited by, among other things, the wavelength of the light. For the greater part of the 20th century this led scientists to believe that, in optical microscopes, they would never be able to observe things smaller than roughly half the wavelength of light, i.e., 0.2 micrometres (figure 1). The contours of some of the cells’ organelles, such as the powerhouse mitochondria, were visible. But it was impossible to discern smaller objects and, for instance, to follow the interaction between individual protein molecules in the cell. It is somewhat akin to being able to see the buildings of a city without being able to discern how citizens live and go about their lives. In order to fully understand how a cell functions, you need to be able to track the work of individual molecules.
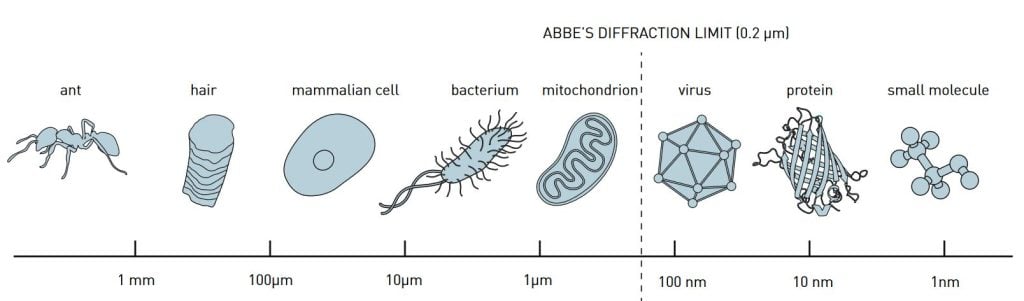
Abbe’s equation still holds but has been bypassed just the same. Eric Betzig, Stefan W. Hell and William E. Moerner are awarded the Nobel Prize in Chemistry 2014 for having taken optical microscopy into a new dimension using fluorescent molecules. Theoretically there is no longer any structure too small to be studied. As a result, microscopy has become nanoscopy.
The story of how Abbe’s diffraction limit was circumvented runs in parallel tracks; two different principles are rewarded, which have been developed independently of each other. We begin in 1993, in a student apartment in South-western Finland, where Stefan Hell had a brilliant idea as he was leafing through a textbook in Quantum Optics.
Youthful revolt against Abbe’s diffraction limit met with scepticism
Ever since getting his Ph D from the University of Heidelberg in 1990, Stefan Hell had been looking for a way to bypass the limitation that Ernst Abbe had defined more than a century earlier. The thought of challenging such an established principle was tantalizing. But senior scientists in Germany had met his enthusiasm with scepticism, and hence Stefan Hell had taken refuge in the cold North. A professor at the University of Turku who was working on fluorescence microscopy had offered him a position on his research team. Hell was convinced that there had to be a way of circumventing Abbe’s diffraction limit, and when he read the words stimulated emission in the book on Quantum Optics a new line of thought took shape in his mind: “At that moment, it dawned on me. I had finally found a concrete concept to pursue – a real thread.” Such was his comment in 2009 – let us delve into his idea.
The solution: a nano-sized flashlight scanning over the sample
In Turku Stefan Hell worked on so-called fluorescence microscopy, a technique where scientists use fluorescent molecules to image parts of the cell. For instance, they can use fluorescent antibodies that couple specifically to cellular DNA. Scientists excite the antibodies with a brief light pulse, making them glow for a short while. If the antibodies couple to DNA they will radiate from the centre of the cell, where DNA is packed inside the cell nucleus. In this manner, scientists can see where a certain molecule is located. But they had only been able to locate clusters of molecules, such as entangled strands of DNA. The resolution was too low to discern individual DNA strings. Think of being able to see a roll of yarn without being able to follow the thread itself.
When Stefan Hell read about stimulated emission, he realized that it should be possible to devise a kind of nano-flashlight that could sweep along the sample, a nanometre at a time. By using stimulated emission scientists can quench fluorescent molecules. They direct a laser beam at the molecules that immediately lose their energy and become dark. In 1994, Stefan Hell published an article outlining his ideas. In the proposed method, so-called stimulated emission depletion (STED), a light pulse excites all the fluorescent molecules, while another light pulse quenches fluorescence from all molecules except those in a nanometre-sized volume in the middle (figure 2). Only this volume is then registered. By sweeping along the sample and continuously measuring light levels, it is possible to get a comprehensive image. The smaller the volume allowed to fluoresce at a single moment, the higher the resolution of the final image. Hence, there is, in principle, no longer any limit to the resolution of optical microscopes.
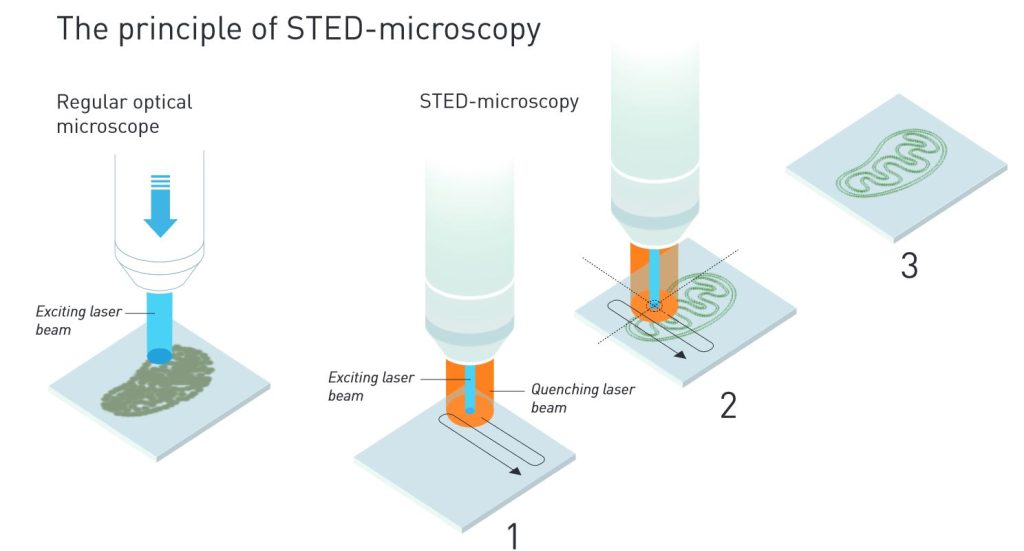
In a regular optical microscope, the contours of a mitochondrion can be distinguished, but the resolution can never get better than 0.2 micrometres.
1. In a STED microscope, an annular laser beam quenches all fluorescence except that in a nanometre-sized volume.
2. The laser beams scan over the sample. Since scientists know exactly where the beam hits the sample, they can use that informa-tion to render the image at a much higher resolution.
3. The final image gets a resolution that is much better than 0.2 micrometre. Illustration: © Johan Jarnestad/The Royal Swedish Academy of Sciences
Developing the first nano-flashlight in Germany
Stefan Hell’s theoretical article did not create any immediate commotion, but was interesting enough for Stefan Hell to be offered a position at the Max Planck Institute for Biophysical Chemistry in Göttingen. In the following years he brought his ideas to fruition; he developed a STED microscope. In 2000 he was able to demonstrate that his ideas actually work in practice, by, among other things, imaging an E. coli bacterium at a resolution never before achieved in an optical microscope (figure 3).
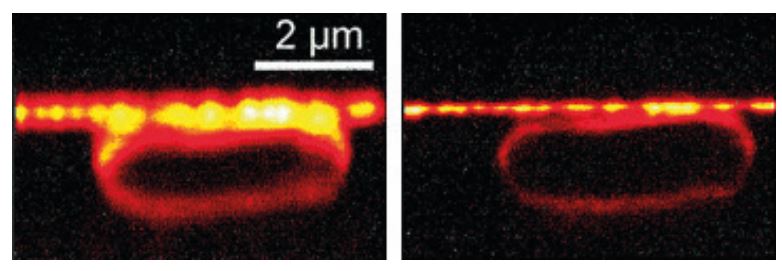
The STED microscope collects light from a multitude of small volumes to create a large whole. In contrast, the second principle rewarded, single-molecule microscopy, entails the superposition of several images. Eric Betzig and W. E. Moerner (who always has been called by his initials, W. E.) have independently of each other contributed different fundamental insights in its development. The foundation was laid when W. E. Moerner succeeded in detecting a single small fluorescent molecule.
W. E. Moerner – first to detect a single fluorescent molecule
In most chemical methods, for instance measuring absorption and fluorescence, scientists study millions of molecules simultaneously. The results of such experiments represent a kind of typical, average molecule. Scientists have had to accept this since nothing else has been possible, but for a long time they dreamt of measuring single molecules, because the richer and more detailed the knowledge, the greater the possibility to understand, for instance, how diseases develop.
Therefore, in 1989, when W. E. Moerner as the first scientist in the world was able to measure the light absorption of a single molecule, it was a pivotal achievement. At the time he was working at the IBM research centre in San Jose, California. The experiment opened the door to a new future and inspired many chemists to turn their attention to single molecules. One of them was Eric Betzig, whose achievements will be covered below.
Eight years later Moerner took the next step towards single-molecule microscopy, building on the previously Nobel Prize-awarded discovery of the green fluorescent protein (GFP).
Molecular-sized lamps turning on and off
In 1997 W. E. Moerner had joined the University of California in San Diego, where Roger Tsien, Nobel Prize Laureate to be, was trying to get GFP to fluoresce in all the colours of the rainbow. The green protein was isolated from a fluorescent jelly-fish and its strength lies in its ability to make other proteins inside living cells visible. Using gene technology scientists couple the green fluorescent protein to other proteins. The green light subsequently reveals exactly where in the cell the marked protein is positioned.
W. E. Moerner discovered that the fluorescence of one variant of GFP could be turned on and off at will. When he excited the protein with light of wavelength 488 nanometres the protein began to fluoresce, but after a while it faded. Regardless of the amount of light he then directed at the protein, the fluorescence was dead. It turned out, however, that light of wavelength 405 nanometres could bring the protein back to life again. When the protein was reactivated, it once again fluoresced at 488 nanometres.
Moerner dispersed these excitable proteins in a gel, so that the distance between each individual protein was greater than Abbe’s diffraction limit of 0.2 micrometres. Since they were sparsely scattered, a regular optical microscope could discern the glow from individual molecules – they were like tiny lamps with switches. The results were published in the scientific journal Nature in 1997.
By this discovery Moerner demonstrated that it is possible to optically control fluorescence of single molecules. This solved a problem that Eric Betzig had formulated two years earlier.
Tired of the academy – but obsessed by Abbe’s diffraction limit
Just like Stefan Hell, Eric Betzig was obsessed by the idea of bypassing Abbe’s diffraction limit. In the beginning of the 1990s he was working on a new kind of optical microscopy called near-field microscopy at the Bell Laboratories in New Jersey. In near-field microscopy the light ray is emitted from an extremely thin tip placed only a few nanometres from the sample. This kind of microscopy can also circumvent Abbe’s diffraction limit, although the method has major weaknesses. For instance, the light emitted has such a short range that it is difficult to visualize structures below the cell surface.
In 1995 Eric Betzig concluded that near-field microscopy could not be improved much further. In addition, he did not feel at home in academia and decided to end his research career; without knowing where to go next, he quit Bell Labs. But Abbe’s diffraction limit remained in his mind. During a walk a cold winter day a new idea came to him; might it be possible to circumvent the diffraction limit by using molecules with different properties, molecules that fluoresced with different colours?
Inspired by W. E. Moerner, among others, Eric Betzig had already detected fluorescence in single molecules using near-field microscopy. He began to ponder whether a regular microscope could yield the same high resolution if different molecules glowed with different colours, such as red, yellow and green. The idea was to have the microscope register one image per colour. If all molecules of one colour were dispersed and never closer to each other than the 0.2 micrometres stipulated by Abbe’s diffraction limit, their position could be determined very precisely. Next, when these images were superimposed, the complete image would get a resolution far better than Abbe’s diffraction limit, and red, yellow and green molecules would be distinguishable even if their distance was just a few nanometres. In this manner Abbe’s diffraction limit could be circumvented. However, there were some practical problems, for instance a lack of molecules with a sufficient amount of distinguishable optical properties.
In 1995 Eric Betzig published his theoretical ideas in the journal Optics Letters, and subsequently left academia and joined his father’s company.
Lured back to microscopy by green fluorescent proteins
For many years Eric Betzig was entirely disconnected from the research community. But one day a longing for science sprang to life again, and returning to the scientific literature he came across the green fluorescent protein for the first time. Realizing there was a protein that could make other proteins visible inside cells revived Betzig’s thoughts of how to circumvent Abbe’s diffraction limit.
The real breakthrough came in 2005, when he stumbled across fluorescent proteins that could be activated at will, similar to those that W. E. Moerner had detected in 1997 at the level of a single molecule. Betzig realized that such a protein was the tool required to implement the idea that had come to him ten years earlier. The fluorescent molecules did not have to be of different colours, they could just as well fluoresce at different times.
Surpassing Abbe’s limit by superimposing images
Just one year later, Eric Betzig demonstrated, in collaboration with scientists working on excitable fluorescent proteins, that his idea held up in practice. Among other things, the scientists coupled the glowing protein to the membrane enveloping the lysosome, the cell’s recycling station. Using a light pulse the proteins were activated for fluorescence, but since the pulse was so weak only a fraction of them started to glow. Due to their small number, almost all of them were positioned at a distance from each other greater than Abbe’s diffraction limit of 0.2 micrometres. Hence the position of each glowing protein could be registered very precisely in the microscope. After a while, when their fluorescence died out, the scientists activated a new subgroup of proteins. Again, the pulse was so weak that only a fraction of the proteins began to glow, whereupon another image was registered. This procedure was then repeated over and over again.
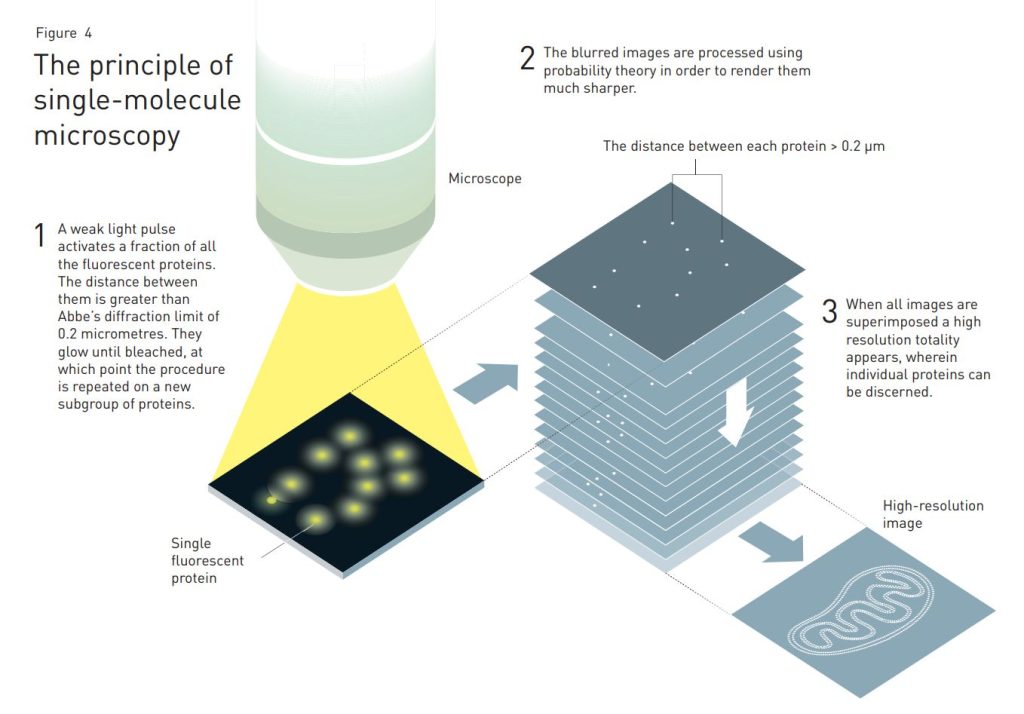
When Betzig superimposed the images he ended up with a super-resolution image of the lysosome membrane. Its resolution was far better than Abbe’s diffraction limit. An article published in Science in 2006 subsequently presented the ground-breaking work.
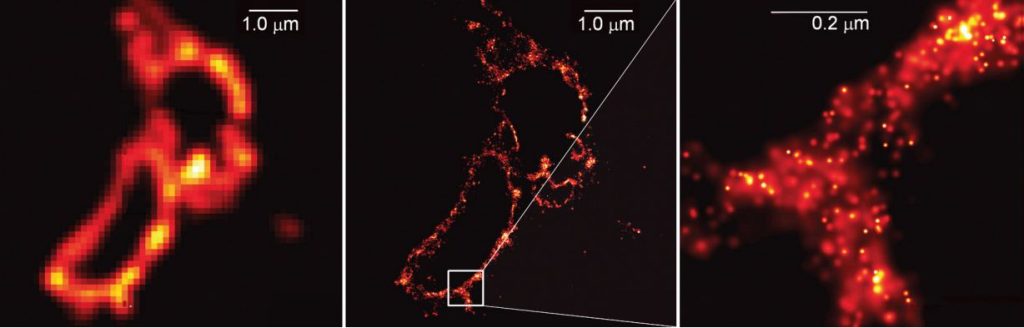
The laureates are still mapping the innermost secrets of life
The methods developed by Eric Betzig, Stefan Hell and W. E. Moerner have led to several nanoscopy techniques and are currently used all over the world. The three Laureates are still active researchers in the large and growing community of scientists spearheading innovation in the field of nanoscopy. When they direct their powerful nanoscopes toward the tiniest components of life they also produce cutting-edge knowledge. Stefan Hell has peered inside living nerve cells in order to better understand brain synapses. W. E. Moerner has studied proteins in relation to Huntington’s disease. Eric Betzig has tracked cell division inside embryos. These are just a few of many examples.
One thing is certain, the Nobel Laureates in Chemistry 2014 have laid the foundation for the development of knowledge of the greatest importance to mankind.
Links and further reading
Articles
Nair, P. (2012) QnAs with W. E. Moerner, Proc. Natl. Acad. Sci. USA 109(17):6357
Deffke, U. (2011) Outsmarting optical boundaries, www.mpg.de/1039625/Optical_Boundaries
Gewin, V. (2006) Eric Betzig, group leader, Janelia Farm Research Campus, Howard Hughes Medical Institute, Leesburg, Virginia, Nature 440:578
Howard Hughes Medical Institute, Biography Eric Betzig, www.hhmi.org/scientists/eric-betzig
Lectures and interviews (video)
Betzig, E. (2012) Day 2 Distinguished Lecture by Dr. Eric Betzig at MF Symposium, www.youtube.com/watch?v=UqMlL8-eaxk
Interview with Betzig, E. (2013) Eric Betzig Sequence, www.youtube.com/watch?v=RfmgDv46sC8
Interview with Hell, S. W. (2013) Stefan Hell Sequence, www.youtube.com/watch?v=WjBPFpVu_6I
Interview with Moerner, W. E. (2013) Alumni Achievement Award: W.E. Moerner
www.youtube.com/watch?v=VBmgvIATbCc
The Laureates
ERIC BETZIG
U.S. citizen. Born 1960 in Ann Arbor, MI, USA. Ph.D. 1988 from Cornell University, Ithaca, NY, USA. Group Leader at Janelia Research Campus, Howard Hughes Medical Institute, Ashburn, VA, USA.
http://janelia.org/lab/betzig-lab
STEFAN W. HELL
German citizen. Born 1962 in Arad, Romania. Ph.D. 1990 from the University of Heidelberg, Germany. Director at the Max Planck Institute for Biophysical Chemistry, Göttingen, and Division head at the German Cancer Research Center, Heidelberg, Germany.
www3.mpibpc.mpg.de/groups/hell
WILLIAM E. MOERNER
U.S. citizen. Born 1953 in Pleasanton, CA, USA. Ph.D. 1982 from Cornell University, Ithaca, NY, USA. Harry S. Mosher Professor in Chemistry and Professor, by courtesy, of Applied Physics at Stanford University, Stanford, CA, USA.
http://web.stanford.edu/group/moerner
Science Editors: Måns Ehrenberg and Sven Lidin, the Nobel Committee for Chemistry
Text: Ann Fernholm
Illustrations: © Johan Jarnestad/The Royal Swedish Academy of Sciences
Editor: Ann Fernholm
© The Royal Swedish Academy of Sciences
Nobel Prizes and laureates
Six prizes were awarded for achievements that have conferred the greatest benefit to humankind. The 12 laureates' work and discoveries range from proteins' structures and machine learning to fighting for a world free of nuclear weapons.
See them all presented here.