Eric Betzig
Biographical
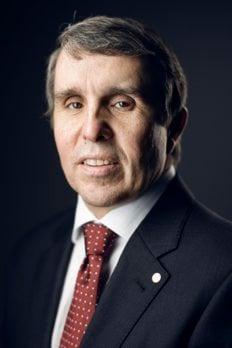
I was born and raised in Ann Arbor, Michigan, a university town. Even though my parents were not affiliated with the university while I was growing up, I think that environment and the people I encountered there really contributed to my latching on to science.
My Dad went to the University of Michigan as a physical education major right after World War II. He became the captain of the wrestling team (Figure 1), and after graduation, he was asked to stay on as an assistant coach. Eventually, to make more money, he started working as a junior draftsman at a machine tool business, and my parents put down roots in Ann Arbor.
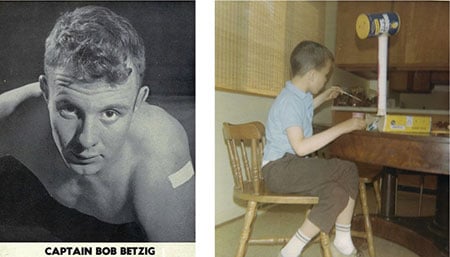
My Dad taught me the value of hard work. He came from very humble beginnings, but eventually started his own company and grew it to 300 employees and 75 million dollars in annual sales. My mom raised my two older sisters, my younger brother, and me. Both my parents were quite intelligent, as well as extremely competitive. My Dad was an All-American wrestler who just missed the 1948 Olympic team. My Mom didn’t have any outlet for her intelligence, and I think she was unhappy being locked into her role as a housewife in the 1950s and 60s. But she loved to trounce all the contestants on Jeopardy when it came on TV. Genetically, I think my parents’ competitiveness coherently interfered in me to create something four times as competitive as either one of them. I don’t like to lose.
I had a happy childhood. I was in Boy Scouts and I’d play a bit with other kids, but nothing really interested me as much as reading and learning. My siblings say I was self-absorbed. I latched on to the space program around the time I was in kindergarten, drawn to the exploration and the excitement, the rockets and the power. I drew elaborate designs of spacecraft and other nonsensical machines that my fifth grade teacher hung in the corridors of the elementary school, and when I was a bit older, I was really into building model rockets. When I was talking about science I could be engaging, but outside of that, I was very shy.
In the third grade, I made friends with a boy whose Dad was a scientist at the university, and he infected me with his enthusiasm for science. We had a subscription to the Science Service, and waited with baited breath for the company to mail us a new kit each month so we could get started building a battery or doing the next experiment. By the end of fourth grade, I had exhausted every science-related book in the library and was looking for more to learn. I remember writing a letter to a scientist at the university right after quarks were discovered. I asked him about the mass and charge of quarks, and I was so excited when he wrote back with answers to my questions. By middle school, I wanted to be a theoretical astrophysicist.
In seventh grade, I’d spend most afternoons messing around with a couple of friends in the science teacher’s back room, which had everything you would need to make everything from fireworks to a Van de Graaf accelerator. We usually stayed until dinnertime, making gunpowder or mixing chemicals to see what colors they gave off when you added various metals. That guy would probably be arrested if he allowed that today, but that freedom to explore was so valuable.
By high school, I became a machine of studying. I took advanced placement everything, whether it interested me or not, and I pulled innumerable all-nighters working on assignments. My attendance was probably barely enough to pass, but man, I learned a lot. My biology teacher senior year was Mr. Young. Knowing he had never given an A, I made it my mission to get an A+ in his class. Every week, we had another complicated and extremely detailed lab, and that’s where I realized I liked experimentation and doing things with my hands. I turned in a 50–100 page lab report every week, and I got the A+.
After high school, I went to Caltech. I went with much trepidation, afraid I wouldn’t measure up to my classmates. I didn’t realize that my public high school was probably one of the best in the country, and it turned out I was better prepared than most of the kids.
I threw myself into my coursework, taking harder and harder classes. By my junior year, I found my limit. It felt like an infinite loop of homework and studying and test taking. I had to take the third trimester off because of my health – my hair was falling out and I had eczema so bad I looked like a lobster. I’ve never worked that hard at any other time in my life.
I got a great education at Caltech but it was extremely theoretical. It was an independent project in a fluid mechanics lab that hooked me on doing experimental research. My advisor, Garry Brown, encouraged me to present my research at an undergraduate competition held by the American Institute of Aeronautics and Astronautics. My practice talk was incredibly dense and full of equations, and he ripped it to shreds. He taught me that when you give a talk, you are telling a story. That was incredibly helpful in learning how to communicate science, and in the end I won the nationals of that competition. That experience convinced me to be an experimental scientist.
So, when I graduated from Caltech in 1983, I wanted to find practical applications of my physics. Cornell offered one of the few applied physics programs in the country at that time, and its student population was 50 percent female. Although I was still very shy, I knew if I went there I would be forced to confront the opposite sex. So I went to Cornell.
The path to the rest of my career was set almost immediately. After the first semester, I met Mike Isaacson and Aaron Lewis. Aaron had been trained as a chemist in Raman spectroscopy, and Mike was one of the first guys to directly image atoms in an electron microscope. He had turned that microscope around and made it into a lithography tool that could make holes as small as 30 nanometers in a thin membrane, which you could then coat with metal to make it opaque.
He and Aaron thought that if they shone light through those apertures and scanned it across living cells, they could illuminate just a 30 nanometer region and produces images with the resolution of an electron microscope. This sounded like it could be something big, and I wanted in.
Once I got started, I couldn’t wait for classes to end. I wanted to be in the lab all the time. The first couple of years were really hard. We had no money. Fellow graduate student Alec Haratounian and I were able to make the patterns at the sub-micron facility, but we had to borrow or build just about everything else. So I learned how to make things work when you don’t have anything to work with. My advisors mostly left me alone, so once we got a grant a few years in, I was free to make my own mistakes. I made plenty of mistakes, but you learn by failing, and I learned a lot.
I did a lot of work characterizing the apertures, and Alec started putting together a test rig. But to make those apertures, the membrane had to be 100 nanometers thick, and if you looked at them the wrong way they would shatter. After the first couple of years, we figured out we could pull glass pipettes like people were doing for patch clamping to get the tips we needed for the probes. Alec got his thesis, and I built this crazy, elaborate, expensive microscope that kind of worked (Figure 2). I never looked at much beyond test patterns, but it was enough to prove that the idea was valid.
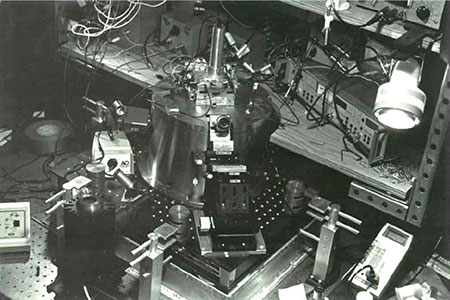
In that era, Cornell was at the forefront of many aspects of cryogenic condensed matter physics, which made it a recruiting conduit to Bell Labs. I wasn’t salivating to go there, but I got an interview and I went to visit in early 1988. By the time I left, I knew it was where I really wanted to be. Everybody was so bright, and they didn’t hold anything back. I had prepared a 45 minute talk and it took an hour and a half because I was just peppered with questions. It was fantastic.
During that visit, I met Horst Störmer, head of the semiconductor physics research department (Figure 3). Horst radiated enthusiasm and energy out of every pore, and he was just brilliant besides. I knew next to nothing about semiconductor physics, but Horst thought near-field was a really cool idea and it could go places. He wanted to hire me, even though what I was doing was completely outside of what everyone else in the department was doing. Except for one guy – Harald Hess, who I also met during that visit. Harald had built a low temperature scanning tunneling microscope to study superconductors, and he and I hit it off immediately.
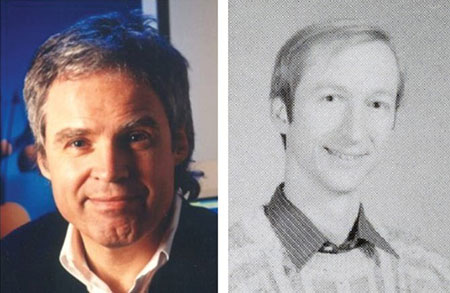
I was hired in Horst’s department, and I picked up where I’d left off with near-field. I had enough resources to build my microscope, but the damn thing wasn’t working any better for me than it did at Cornell. Because of Bell Lab’s history and the brilliance of everyone around me, I felt like I was on probation from the time I got there. Two years in, I wrote in my self-evaluation that if I didn’t have a breakthrough in the next year, they wouldn’t need to fire me because I would quit.
Harald really kept me afloat both personally and professionally during that time. We both worked insanely hard during those years. I would come into work at 4:30 in the morning, and if I saw Harald’s car, I would put my hand on the hood to find out if the engine was still warm. He did exactly the same thing. We were both really competitive, but we played tennis every morning and ate dinner together every night. We were best friends and still are.
During my third year, I stopped just trying one thing after another, and started thinking like a physicist about why things weren’t working. Once I understood the physical problems of the pipette tip I was using, I realized I could replace it with an optical fiber, which would deliver more light to the tip. I also came up with a method of using dissipation force feedback to regulate the tip’s distance from the surface of a sample, which didn’t break tips like my old method and could be used on cells as well as semiconductors.
With those breakthroughs, the next few years were the golden age for nearfield micoscopy. I tried the technology everywhere I could think of. Sometimes it worked and sometimes it didn’t, but the papers came quick. In 1992, we applied it to data storage – at one time we held the world record for storage density – and in the following year I demonstrated super-resolution fluorescence imaging of cells for the first time.
W.E. Moerner had been the first to see the spectral signature of single molecules at cryogenic temperatures in 1989, but no one had yet imaged single molecules at room temp. You needed focused light, because otherwise the background would obscure the signature of a single molecule. At the time, there was no better means of focusing light than near-field microscopy, and as soon as I attempted that experiment, it worked. Surprisingly, the molecules looked like arcs instead of round spots – the molecule was acting as the light source, and its dipole moment was mapping out the electrical field inside the near-field aperture. It was an afternoon experiment that was a shock on many fronts.
For my last hurrah with near-field, Harald and I put my near-field probe on his low-temperature tunneling microscope to study quantum well structures, which are the basis of semiconductor lasers, like those in laser pointers. With standard diffraction-limited optics, their spectrum looks like a smooth hill of emission, but we saw a crazy series of super sharp lines. Our probe volume was so small, the light could only be emitted at certain discrete sites. And the wavelength of that light was very sensitive to the local thickness of the quantum well, so they glowed in different colors, which meant we could study them individually.
That was a stunning paper, but by this time I was fed up. Although nearfield has proven to be a valuable tool for materials characterization and studying light-matter interactions at the nanoscale, my original goal was to make an optical microscope that could look at living cells with the resolution of an electron microscope. But near-field only worked on samples that were ridiculously flat, where the thing that you wanted to see was ridiculously close to the surface. If you’re 20 nanometers away, you lose significant resolution. I knew a cell was a bit rougher than 20 nanometers, so it just wasn’t going to happen.
Meanwhile, the field had blown up. There were hundreds of people doing near-field by this time, and much of it was crap. People were fooling themselves with images that had sharp-looking but artifactual structures, and they just didn’t want to hear it. I felt like every good result I had provided justification for a hundred lousy papers to follow, and that was a waste of people’s time and taxpayers’ money.
On top of that, it was clear by 1994 that Bell was coming to an end. The phone monopoly had been broken up in 1984, and it was hard for AT&T to justify all the spending on basic science. We could feel the weight of the world on our shoulders. I was exhausted.
So I quit. While my wife worked, I stayed home with our daughter Kriya, who was born in 1993, and became a house husband. I really didn’t know what I wanted to do.
My Dad had worked for the same company until he was 60, then used his retirement savings to start a competing company. He had always wanted me to come work for him. So I made occasional trips to Michigan to consult for him while I raised my daughter in New Jersey and continued thinking about science.
A few months after I left Bell, I was pushing Kriya around in the stroller and it popped into my head that if you could somehow isolate different molecules in multiple dimensions, you could localize each one and do super-resolution imaging that way. I was excited about this for a couple of months, and I published the idea. But I knew using it for biology was going to be very difficult, because there could be hundreds or thousands of proteins in a single diffraction-limited spot, and there was no easy way to distinguish them at room temperature. As an engineer, I didn’t want to do a hero’s experiment; I wanted to do something useful.
When Kriya was three, and started speaking with a Jersey accent, I knew we had to get out of New Jersey. I eventually became convinced from the consulting I did that I could have an impact in my Dad’s company, so we moved to Michigan in 1997 and set down roots.
My Dad gave me as much freedom as I had at Bell. My proudest development was a servo-hydraulic machine tool that combined old hydraulic technology with modern control theory and the energy storage principles that are in today’s hybrid cars (Figure 4). It could move four tons at eight Gs of acceleration and position to five micron precision. It was much smaller, much cheaper, much faster, and much better than any previous technology. It was also too different, and after three or four years developing it, I couldn’t sell it worth beans.
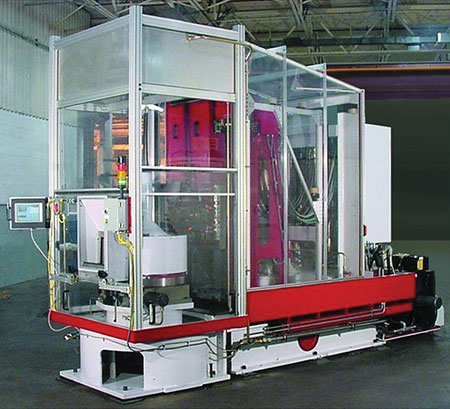
You work under so many more constraints in the business world than you do in academia – anybody who can make a profit has nothing but my utmost respect. But I was very bad at it, and I felt like I was only using a small fraction of what I knew and was good at, which was physics. So in 2002 I quit.
That was probably the hardest part of my life. I had pissed away my academic career and I had pissed away my backup plan of working for my Dad. Once again, I didn’t have any idea what I wanted to do. Fortunately, with money in the bank, I had some time to think of a solution.
I reconnected with Harald, who had also gone into industry in San Diego, but wasn’t completely satisfied. We started meeting in various national parks (Figure 5) and confronted our respective mid-life crises, asking: What do we want of life? What’s important? How can we have an impact? The rest of the time, I would go to a cottage we had on a lake in Michigan to think while Kriya and her brother Ravi were in school.
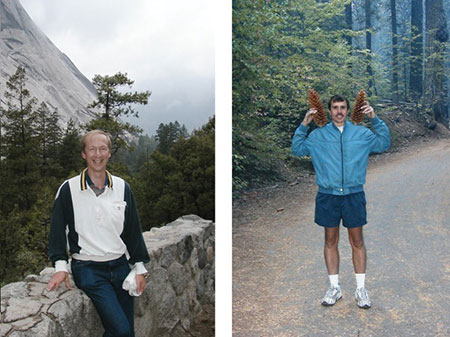
I started reading the scientific literature again, and quickly came across Marty Chalfie‘s paper on green fluorescent protein, which he had published in 1994 as I was leaving Bell. It was like a religious revelation to me. Part of what made imaging with near-field so difficult was that it was hard to label proteins densely enough without also putting the fluorophore on a bunch of nonspecific crap. Here was a way to label with 100 percent specificity, and you could do it in a live cell. I couldn’t believe how amazingly elegant it was. I hadn’t wanted to go back to microscopy, but once I learned about GFP, I felt like I had to.
I wanted to take advantage of GFP to do live cell imaging, but my physics knowledge had atrophied. So I pulled out my old textbooks and started redoing old homework problems. I was really motivated to understand it this time around, because I figured this was my last chance to make a scientific career. The knowledge was all in my head, it was just kind of stuck behind a wall, and that wall came down quickly. Robert Heinlein in Stranger in a Strange Land has this word called “grok,” where you know something so well that you love it and hate it and it’s part of you. Within three months, I grokked diffraction and light and formation of foci.
I started to think about using multiple foci to image more quickly. I learned about optical lattices, which had been developed several years earlier, and came up with a theory for new lattices that would allow for faster, less damaging imaging. After thinking through all the potential applications, I filed a patent that was more than 300 pages long.
I tried to convince Harald to come make this lattice microscope with me. He was interested, but unsure. I also contacted Horst, who had won the Nobel in 1998, and was now at Columbia. He invited me to present the idea to the biology department there in April 2005. Marty Chalfie was one of my hosts during that visit, and he turned to me in the cab on the way to dinner and said, “It sounds like you really believe in this idea. How are you going to get back in the lab?” I said, “I have no idea, but I read in Physics Today that there’s a guy named Gerry Rubin who wants to make a biological Bell Labs,” and we left it at that.
I stayed focused on finding a way to do the lattice microscope, and in the same month went with Harald to meet Mike Davidson at Florida State University. Mike had one of the biggest libraries of fluorescent protein fusions in the world, and that’s where we learned about photoactivatable fluorescent proteins. In the Tallahassee airport on our way home, it became obvious to Harald and me that this was the missing link for the idea that I had pitched after I left Bell: we could isolate a few molecules at a time by activating limited subsets of photoactivatable proteins. It seemed so easy. We immediately abandoned the lattice idea and started writing claims for patents. We continued to meet in various national parks, and planned our research and patent strategy.
Harald and I didn’t know any biology, so we needed help. I arranged to meet the developers of the photoactivable fluorophores, Jennifer Lippincott-Schwartz and George Patterson at the National Institutes of Health and told them our idea. Jennifer told us to build the microscope and bring it by.
Harald and I built the first PALM microscope in his living room in La Jolla (Figure 6). We were both unemployed, but Harald had some of his equipment from Bell. We pulled that out of storage, and each put in $25,000 to cover everything else we needed. We worked hard, and in September shipped all the parts to rebuild the microscope in the darkroom of Jennifer’s lab at the NIH. The first time we put a cover slip coated with molecules into the microscope and turned on the photoactivating light, the first subset popped up and we knew we had it.
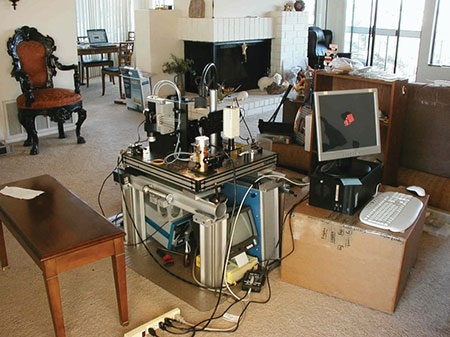
By limiting the photoactivating light so that only a few labeling molecules appeared in each image, we could find the center of each spot. Repeating this 10 or 20 thousand times built up the super-resolution image. By early 2006, we had 20 nanometer resolution images of actin filaments, focal adhesions, mitochondria, and lysosomes. We submitted the work to Science in March, and it was published that August, after a lengthy fight with a reviewer who demanded correlative EM data, and then pushed for rejection even after we supplied it.
Meanwhile, Marty had told Gerry that I was interested in that “biological Bell Labs,” HHMI’s new Janelia Farm Research Campus. The campus wasn’t built yet, but I was invited to interview in a little building off site in August, and was on the payroll in October 2005.
Once the building opened in 2006, postdoc Hari Shroff and I lived and breathed PALM for the next few years. It was a very competitive time in super-resolution. We developed multicolor capability, and demonstrated live-cell PALM. We also developed with Jennifer a method to study cellular transport by watching subsets of molecules diffuse in a cell, and we had a few other successes here and there.
In 2008, Nature Methods named super-resolution “Method of the Year.” Everyone and his kid sister were doing super-resolution by that time. Just like when near-field was at its peak, people were making all kinds of claims that I knew were impossible. Although we had demonstrated live cell imaging, PALM is too slow and throws too much light at a sample to be a practical solution for that. The field was getting crowded, and I’ve always found it most productive to go where the people aren’t. It was time to do something new.
Many of my neuroscientist colleagues at Janelia were trying to peer inside the brains of flies and mice, and I knew that imaging gets pretty crappy when you try to look deep below the surface of the tissue. We needed adaptive optics to correct for distortions caused by heterogeneity of the tissue. Astronomers deal with this problem in telescope images by shining a laser high in the atmosphere in the direction of the object they are observing, then measuring with a special sensor how the light from that guide star is distorted as it returns to Earth. We couldn’t use quite the same approach because scattering in the brain obscures the guide star, so in 2010 postdoc Na Ji and I turned the sensing principle on its head and used image displacements in the sample itself as the sensor. Na has since improved this idea greatly in her own lab, and uses it to record neural activity deep in the cortex with much greater accuracy and reliability.
Meanwhile, Ernst Stelzer had come to Janelia in 2008 and spoke about using a sheet of light to image a single plane at a time within a specimen while avoiding illuminating the regions above and below. I thought that was an elegant solution to the problem of photodamage, and wanted to contribute something new. A light sheet is typically too thick to see detail inside of cells, so with postdocs Liang Gao and Thomas Planchon, we used something called a Bessel beam that we scanned across the sample to create a much thinner light sheet. Within a year or so, we were imaging dynamics within living cells with good resolution in all three dimensions.
One of the problems we encountered was that the Bessel beam had side lobes of weaker light, which created out-of-focus excitation. Liang eventually overcame that problem by stepping the beam instead of sweeping it, and using structured illumination microscopy (SIM), originally developed by my Janelia colleague Mats Gustafsson, to exploit the resulting periodic excitation pattern and extend the resolution a bit beyond the diffraction limit in two of the three dimensions.
To not sacrifice speed when stepping the beam, we generated seven Bessel beams in parallel. To our initial surprise, spreading the energy seven-fold significantly cut the photodamage. What we learned was that while the total dose of light you put into the cell is important, what’s far more important is the instantaneous power delivered to the cell. I then realized that this was consistent with what Na and I had found earlier in 2008 when we reduced the damage associated with two-photon imaging by splitting ultrafast light pulses into a series of sub-pulses of much lower peak power.
Why stop at seven? I modeled the interactions of additional beams, and found that as they become crowded and the side lobes start to interfere, you get crazy resonances and anti-resonances – but there are magic periods where all of a sudden the side lobes destructively interfere. It’s a triple win: You spread the energy out, get a very thin light sheet by eliminating the nasty side lobe problem, and you create a high contrast light sheet ideal for SIM.
That brought me back full circle to the optical lattice theory I had published in 2005. That theory predicted exactly what types of light patterns would create these magic periods. Postdoc Kai Wang figured out how we could use a spatial light modulator to produce these patterns in the lab, and my other postdocs Bi-Chang Chen and Wesley Legant built lattice light sheet microscopes to discover what we could do with this technology.
As it turns out, a lot. The light sheet is so thin that only in-focus molecules are illuminated, making it the perfect tool to push all single molecule imaging methods, including PALM, past their previous limitation to thin samples. Ditto with SIM. When used in a diffraction-limited mode, we can often record several image volumes per second or, at slower speeds, image many brightly labeled samples indefinitely. We worked with over thirty different groups on everything from the kinetics of single transcription factor molecules in stem cells to cell division, 3D cell migration, and embryonic development before publishing the method in Science shortly after I received the Nobel.
I think the super-resolution field is still sorting itself out, but I have a suspicion that the lattice light sheet microscope, and not PALM, will be the high water mark of my career. I’ll never be a biologist, but I get a kick out of the beauty of the movies, the craziness of the cell, and the opportunity to learn from dozens of the best biologists in the world. Every week it’s a new adventure.
Mats passed away in 2011, but we also continue to push the limits of SIM. My postdoc Dong Li has extended live cell SIM to 50 nm resolution at subsecond frame rates. Because it is so much faster and uses so much less power than PALM, STED, or RESOLFT, I think there’s a good chance that SIM will be the super-resolution method that will have the greatest impact in live imaging.
I feel like I’ve been incredibly lucky to have had the career I’ve had. Everywhere I’ve been, I’ve been able to focus 100% on my work – I’ve never written a grant in my life. I doubt I would have been as successful in a more traditional academic career path. My group at Janelia has never been larger than five postdocs, and has averaged three. It’s tremendous fun to be able to work closely with them, and it’s exciting to feel like I have a real intellectual stake in what comes out of the lab. I doubt I’d have the same rush with a larger group such as is common elsewhere. In fact, I think that our research model gives us an almost unfair competitive advantage over our peers.
I’m also lucky in that I have a second chance to be a better husband and father. While I’m close with Kriya and Ravi, one of my regrets is that I didn’t spend more time with them when they were growing up. Na and I have two happy and beautiful little hellions, Max and Mia, and I have the opportunity to be with them more. I don’t know, though, if I’ll ever figure out how to optimally balance my responsibility and desire to be at both work and home.
Being fundamentally a pessimist, I still have two fears. One is that the distractions from the Nobel will disrupt our research model and hamper our productivity, as it has already begun to do. The other is that I feel we’ve been too successful. There’s important work still to be done, such as in a project by my postdoc Tsung-Li Liu to combine an adaptive optics method for transparent specimens developed by Kai with the lattice light sheet tech developed by Bi-Chang and Wes. This would allow us to take cells away from the cover slip, and place them back into the multicellular environment in which they evolved. However, this too is likely to succeed. I think it’s my obligation, given the resources at Janelia and the prestige and security of the Nobel, to throw the dice again, and do crazy, risky stuff. Harald and I are working together again with our respective groups in this direction. Only time will tell if anything comes of it, which is just the way I like it.
This autobiography/biography was written at the time of the award and later published in the book series Les Prix Nobel/ Nobel Lectures/The Nobel Prizes. The information is sometimes updated with an addendum submitted by the Laureate.
Nobel Prizes and laureates
Six prizes were awarded for achievements that have conferred the greatest benefit to humankind. The 12 laureates' work and discoveries range from proteins' structures and machine learning to fighting for a world free of nuclear weapons.
See them all presented here.