John B. Goodenough
Biographical
Childhood
I was born in Jena, Germany, in July 1922, to American parents, Erwin Ramsdell Goodenough and Helen Miriam (Lewis) Goodenough. My father was working on his D. Phil. dissertation on the Church Fathers at Oxford University at the time of my birth. My parents lived in Oxford, England, for three years and my father enjoyed the culture of the Weimar Republic; he spent much of his long summer vacations in Germany as well as in Rome. My grandfather was able to support my father before the market crash of 1928. After my parents returned to the U.S. from Oxford, my father became a professor of the history of religion at Yale University. My grandfather Goodenough had bought for him with a large mortgage an old house on a five-acre lot complete with an adjoining woodshed, a large barn, an ice house, and a windmill for pumping our water from a spring in the back lot. A huge wisteria vine on which we could climb was supported on the south side of the house by a giant maple tree and on the east side by a trellis. On the north side, a U-shaped driveway leading past the house to a barn encircled apple trees. The windmill, which invited young boys to climb it, was then quickly replaced by an electric pump and the coal-fired furnace by an oil furnace. However, ice was still hauled to an icebox and kerosene for the stove was fetched from a little house on the north border of the property. A large veranda in the front of the house faced west overlooking a two-bar fence before a row of elm and maple trees bordering Amity Road, the main bus route between New Haven and Waterbury. We were located seven miles north of Yale University, which is in downtown New Haven.
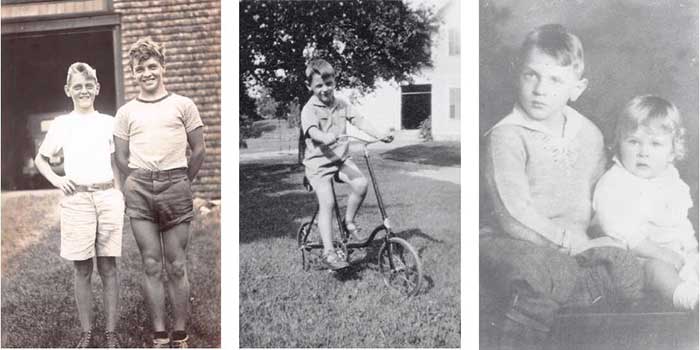
Figure 1. Left: My older brother Ward (right) and I (left) outside our house in Woodbridge. Middle: With my bicycle circa 1930. Right: My younger sister Hester (right side) and I (left side).
My older brother, Ward, and I shared the north bedroom. Ward was the leader; I was the tag-along when he would tolerate me. My world was with my dog, Mack, in the nearby meadows and woodlands where there was so much life to discover and so much wonder to experience. I liked collecting trophies, whether it was butterflies, seashells, or animal skins. A special room halfway up to the hayloft in our large barn was where I housed my skin and hawk-wing collection. Despite many pleasures that I experienced throughout my childhood, my years through 7 were difficult for me. How I struggled to learn to read! I read mechanically without the ability to catch easily the meaning of a paragraph. I never was a good reader, and through my school years, I worked hard to cover my deficiency. I also had a deep sense of insecurity that only lifted slowly as I grew older.
Early education
I went to a private grammar school in downtown New Haven about a mile from my father’s office in Jonathan Edwards College at Yale University.
My father drove me to school on his way to work. I went with a lunch pail; the meal at school was too expensive. I didn’t mind eating separately. I made friends easily as I enjoyed sports on the playground and being mischievous with the other boys in the back row during French class.
I arrived with a full scholarship to Groton School on a warm September day in 1934. I was shown my cubicle in the Hundred House dormitory; each student had a bed, a dresser, and a place to hang his suits. Suits and neckties were worn at all times but on the athletic field; a stiff collar and patent-leather shoes were worn to dinner. On the first floor of the dorm, there were assigned desks in the study hall for First and Second Formers; Third Formers had a study hall in Brooks House at the other side of the chapel. Older boys had personal one- or two-boy studies.
The School day was regulated by bells. A School House bell rang out across the Circle at 7:00 a.m. After breakfast, the school day began with a 15-mintue chapel service at 8:15 before the trek to our homeroom desk. First Formers had their own homeroom; all others had desks in a large study hall where announcements were made after morning classes before being dismissed to Hundred House for lunch. Afternoons were for doing homework in a study hall for an hour followed by mandatory participation in sports until supper. The Rector kept boys occupied every hour of the day. The only exception was Sunday, a day for writing home, attending chapel morning and evening, and reading in the library.
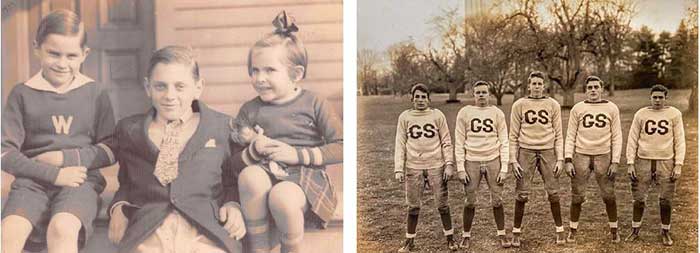
Figure 2. Left: My siblings Hester (left), James (right), and I (middle) in 1933. Right: With my classmates on the Groton School football team in 1939; I am second from the right.
Each class, with the exception of First Form and Sixth Form Sacred Studies, was assigned three sections: B, A, and upper A. I entered as a B student in all subjects. Even so, I was overwhelmed the first few weeks. The Rector taught First Form Sacred Studies. On our first class test, we were asked to name the 12 disciples of Jesus. As I was a poor reader with no religious training, I think I remembered no more than one. Latin class with the football coach went no better, but English grammar under Zahner turned out to be a memorable learning experience. In my Fifth Form year I had been advanced from the B through the A to the upper-A division in all my classes, and I managed to graduate magna cum laude at the head of my Form.
Little time was spent in New Haven after I left home for School. Invitations to spend days or weeks with classmates were common. One summer we were in Colorado and others were mostly relieved by working as camp counselor and lifeguard. I was 14 when I first became a counselor at a YMCA camp. The summer I turned 17, my father added $150 to my savings to allow me to go to Finland with a group from Putney School.
Undergraduate years
I entered Yale University as an undergraduate student in 1940. As I left home, I determined never again to take money from my parents; I had no idea how I would support myself at Yale, but my focus was to do well in my College Board Exams. Fortunately, I was given a summer job tutoring a grandson of the Rector; it would give me room and board for the summer and enough money to pay for a room in a dormitory at Yale the following year.
My freshman year at Yale I was permitted to take a junior course in Ethics and Aesthetics in lieu of English; a junior course translating the Greek plays, and a sophomore second-year calculus course also gave me a good start. My freshman chemistry, Qualitative Analysis, was to satisfy my science requirement and to give me the possibility of Medical School. Freshman Psychology proved to be an intellectual insult; I found Freud totally unconvincing in many of his assertions, and Pavlov’s dog seemed to be more trivial than made out. I was not drawn to the behavioral sciences even though Psychiatry was quite the in-subject at the time.
A common temptation of youth is the desire to be famous or glamorous or powerful. I realized that not everyone can be “king of the mountain” even for a short time. Can being king, therefore, be what gives meaning to life? I began to understand that any meaning to a life is not to be king of a castle, but the significance and permanence of what we serve. Is service to ourselves, our tribe (ethnic group or family), or to our country the highest service? In a time of war against evil, service to the cause of a more just world and therefore to our war effort was meaningful; but the destructive means of war is always an appalling waste on all sides. I struggled to find a meaningful calling beyond the war. Perhaps it would be in science; so, for my sophomore year, I decided to enroll in the philosophy of science and in physics.
In the summer of 1941, I tutored the son of a Chicago banker who lived in Wheaton, Illinois. Their home was a grand mansion built by the grandfather who had worked his way up to become Head of the First National Bank of Chicago. I slept on a porch with the two boys and three great Danes; I was treated as one of the family, and I earned enough money to pay for my room in Timothy Dwight College of Yale. During my latter years at Yale, a bursary job gave me 21 meal tickets during weeks when classes were in session; I was a grader of Freshman mathematics papers. But how was I to afford food during the vacations? Fortunately, the mother of my roommate, Stuart Little, would invite me to Hartford, Connecticut, to share part of my holiday there.
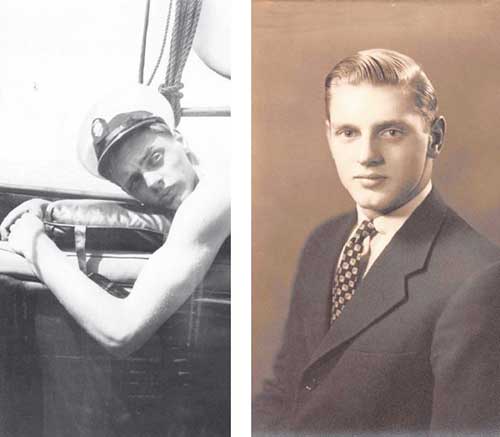
Figure 3. Left: Asleep on a sail up the New England coast. Right: Formal portrait of myself as an undergraduate student in 1942.
When I went to enlist in 1942, my mathematics professor, Egbert Miles, called me into his office and said, “John, don’t sign up for the marines like all your friends. The military needs boys with backgrounds like yours to sign up for meteorology in the U.S. Army Air Corps.” I had no stomach to play the hero in war, so I acted on this friendly advice. It gave me another year in college to finish my undergraduate degree, and I spent the summer of 1942 in New Haven. I was not called to active duty until February of 1943. At that time, I lacked one course for graduation, and Yale graciously gave me credit for my Army course in meteorology to grant me a summa cum laude Bachelor of Arts degree in mathematics in the spring of 1943. However, I was cognizant of the fact that, after completing successfully a second-year physics course, I had been saved from an embarrassing flunk of my first test in the Theoretical Physics course taught by Margenau by a telegram the night before calling me to active duty. I was pursuing physics after reading “Science and the Modern World” by Alfred North Whitehead. While reading that book one evening, it seemed to me that much of the intellectual ferment of my generation would be in science; and physics provides a fundamental foundation for science. If there was to be an opportunity to go to graduate school after the war, I felt that night that I should study physics.
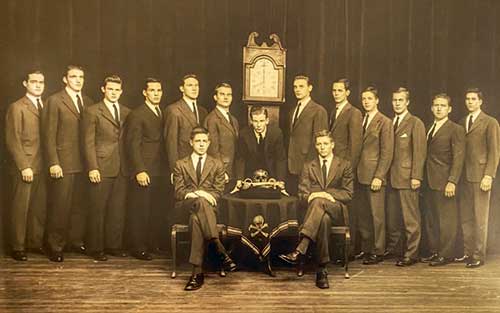
Figure 4. With the members of the secret Skull and Bones society at Yale University in 1942; I am the first person to the right of the clock in the back row.
World War II army meteorology
Entering the Army ended the pressures I had felt at Yale. The struggles to support myself, to find a calling and to finish my undergraduate requirements before being called into the Army all fell away. Most members of my Yale class were at different stages of entering the armed forces when I left, so I was not parting from a normal college experience. Adjustment to military life was not a problem for me. After a brief orientation in Boca Raton, Florida, I went by troop train to Grand Rapids, Michigan, for training to be a practicing meteorologist. The Army training, mostly by civilians, was efficient and quite professional.
Upon commission in the autumn of 1943, I was immediately posted at an air base in Houlton, Maine a few miles south of a more active air base in Presque Isle. Fighter planes were being dispatched from Presque Isle to England. After two weeks, I found myself in charge of the weather station in Houlton. In those days we drew our own maps and made our own forecasts; there was no satellite and no computer-aided forecast from Washington.
Maine proved to be a good training experience for my next posting the following summer in Stephenville on the west coast of Newfoundland. Stephenville was the jumping-off base for the cargo B54s flying to either the Azores, the base of the northern route, or directly to England. These planes also stopped in Stephenville on their way home to Washington, D.C. The B54s had a longer range than the fighter planes. The tactical bombers were dispatched from Gander on the east side of the island.
Although almost all my forecasts were reasonably accurate, including a clearing of Eisenhower from Stephenville that landed him safely in Paris within 6 minutes of his estimated time of arrival, a forecast could be dangerously wrong.
As D-day approached, we tried to predict from the weather when the allies would storm the beaches of France. Eisenhower and our forces had bad luck with the strength of the cold front behind which they attacked. We followed closely the battle of the hedge rows and the final breakout across France.
One December day, civilian pilots flying the B54s were congratulating themselves that they were going to make it home for Christmas. When I refused to clear them for the trip to Newfoundland because a strong headwind from there to the Azores would prevent them from reaching their destination, they set out anyway. Six hours later they were back on base; the headwinds were so strong they had barely cleared the islands.
At the end of the war in Europe in 1945, my thoughts began to return to my struggle with a Christian commitment. In Sta. Maria, a Lutheran Chaplain bowed his head to give thanks before eating, and that simple act in the Mess Hall stirred up into consciousness questions I had suppressed since leaving civilian life. I decided I should read the Bible to let it speak for itself; honest dialogue surely was where I ought to begin. However, life on the base was not conducive to this discipline; the fantasies of youth and the comraderies of my fellow soldiers usurped my leisure attention. With the surrender of Japan, our thoughts turned to the question of how to return to civilian life. A letter from headquarters invited me to stay in the Army as a meteorologist; I was then a Captain. However, I thought our responsibilities in peacetime would be less than those we had assumed in war, so the invitation was declined.
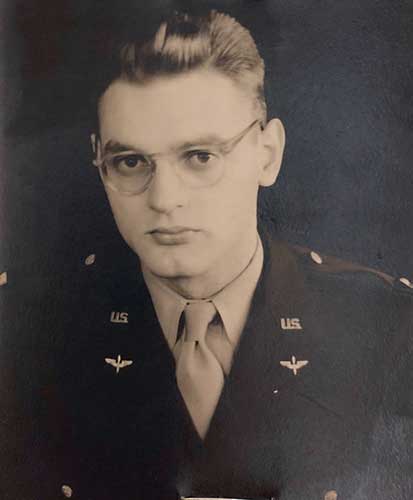
Figure 5. My photograph for University of Chicago International House application when serving in Army during WW II.
Although Law did not attract me as a profession, I entertained the possibility of studying to become an international lawyer. As this idea was developing in my mind, I received a TELEX in the spring of 1946 to return from the Azores to Washington, D.C., within 48 hours. I packed up my duffle bag with great excitement; my turn to go home had come, and I was to embark on a new adventure!
In Washington, I was told that I was one of 21 returning officers who had been chosen to do graduate study in either Physics or Mathematics at the University of Chicago or at Northwestern University; we would remain in-grade, but under the command of the Quartermaster Corps. My mathematics professor at Yale, Egbert Miles, had not forgotten me! His act was unusual; except for him, I was to return as an unknown to begin life all over again. My debt to Professor Miles is profound. He it was who put my name forward when some educators became aware that a sum of unspent money was available; Egbert Miles thought it would be best spent reintegrating a few promising scholars to civilian life by giving them an opportunity to go to graduate school. From Washington I was to go immediately to Chicago as I should have been there 24 hours earlier. That night, on my way to Chicago, a vivid memory returned; I saw myself reading “Science and the Modern World” by Alfred North Whitehead the day I decided I should study Physics if I ever had the opportunity on my return from the war. This opportunity was here! I felt called to sign up for Physics at the University of Chicago the next day even though I believed I would not qualify if they tested my aptitude for the subject. When I went to register, Professor Simpson said to me, “I don’t understand you veterans. Don’t you know that anyone who has ever done anything significant in physics had already done it by the time he was your age; and you want to begin?!” But my decision was made. I had decided to study physics at the University of Chicago.
Graduate years
After serving in the US Army as a meteorologist in World War II, I went to the University of Chicago to do graduate study in Physics from 1946 to 1951. The University of Chicago is located a few blocks from Lake Michigan on the south side of the city; it borders an open strip of grass, called The Midway, that separates 55th street, which runs west from the lake. On the north side of The Midway between the campus and the park was International House; it provided rooms and meals for graduate students and visiting scholars, men in one wing and women in the other with a common room and other facilities between and beneath the dormitories. I managed to secure a room there from the autumn of 1946 until I left Chicago in 1951.
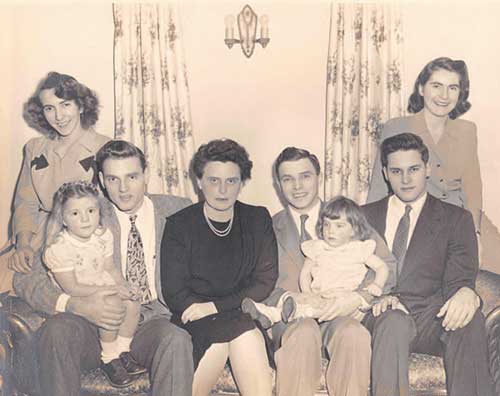
Figure 6. From left: Ruth (Ward’s wife), myself, my mother, Ward (my older brother), James (my younger brother), Hester (my younger sister), and two of my nieces (Ward’s daughters).
Under president Hutchinson, the University of Chicago had a two-year undergraduate program followed by entrance into an Upper Division for graduate study. Unlike in England, where the Advanced (A) Levels before university provided the grounding for a university specialty, these first two years at Chicago were designed to provide a broad liberal education for intelligent citizenship. This arrangement was a perfect match for me. I had not majored in science at Yale but had entertained many subjects in search of a general training. However, students entering the physics program in 1946 were coming from very diverse backgrounds. Almost all had been physics majors, and many came with considerable experimental experience from service assignments at Los Alamos or the MIT Radiation Laboratory. Moreover, the Department was a bit overwhelmed by the number of students they had felt obliged to admit to the program.
My first textbook at Chicago was a tome on mechanics presumably designed some years before 1939 to help students in Cambridge, England, to pass the tripods with the help of a tutor. The first 10 pages so intimidated me that the book was quickly discarded! However, I had the good fortune that first semester to have professors who endeavored to communicate the fundamentals. In the spring of 1947, an examination covering the material of the first year was introduced for the purpose of reducing the class size by 50%. I had done well enough my first year to be exempted from this exam.
Later years proved more difficult. The Physics Department had decided to adopt the Oxford-Cambridge British system of self-study, but without tutors! Lectures covered aspects of a subject that interested the professor; the student was expected to develop on his own the context for the topics covered. Class tests were not necessarily on the lecture topics. Edward Teller, for example, only appeared for three lectures the entire semester I took his class. Moreover, texts on modern physics appropriate for students were scarce or not available. In my course on electromagnetic theory, the text used one set of units, the lecturer another, and the exams were in a third. Although Quantum Mechanics was being developed in the 1920s and 1930s, the war had prevented the appearance of a good text for beginning students. It was easy, therefore, for the student to become so absorbed with the mathematical derivations that the physics was lost. Fortunately, Enrico Fermi introduced us to quantum mechanics. His class on nuclear physics covered ideas developed during the war for which there was no text. His lectures seemed clear enough, but when I attempted to solve his assigned problem for the day, I often found that he assumed we already had the background needed to appreciate fully his expositions. Three of the veteran students developed his lectures into a text that was later used widely. There was no electrical engineering at Chicago. When I asked for a course that would teach me electronics, as I needed to build experimental equipment, I was told to go read the literature. Veterans who had been trained in electronics during the war had an advantage for developing into experimentalists.
At the end of four years, we took a 32-hour written examination on four successive days. Students were only told that the examination would cover all aspects of physics and pertinent topics in mathematics and chemistry. The first eight-hour day consisted of about 32 shorter questions; the second, eight more difficult questions. On the third day there were only four questions, two experimental and two theoretical; the student was to answer three of them. On the fourth and final day there was only one question. The student was allowed to use the library to answer this one. For example, one such final problem was to write a proposal for funding an experiment that required design of a bathysphere for a deepocean study, a defense of the scientific significance of the study, and a design of the instruments to be used to accomplish the desired measurements. The first time I took this examination, I did well enough to be granted an MS degree, but I would have to take it a second time to be allowed to go on for a Ph.D. On my second try six months later, I was so discouraged after the third day that I almost didn’t sit the fourth. I went out and played a game of softball that evening. More relaxed afterwards, I decided I had nothing to lose if I went for the fourth day. I have never really understood why they allowed me to go on for the Ph.D. degree. Only 10% of those with whom I entered the Department in 1946 had made it through this hurdle. In subsequent years, the Department changed this procedure; the veterans and foreign students that entered in 1946-1948 were more mature than those that followed. I knew that I had been exposed to the challenges and practice of the physics profession at its highest level; but for me it was a challenge indeed!
The Physics Department of the University of Chicago also had a policy that no professor was to attach his name to the publication of work reported in a Ph.D. dissertation. Work with the professor as a Research Assistant could not be part of a Ph.D. dissertation. The objective was to ensure that the Ph.D. dissertation represented original research developed and executed only by the student. I knew that I didn’t want to go into nuclear physics, so I opted to do my research in solid-state physics. Professor Clarence Zener was the obvious choice. Zener was involved in the physics of metals, so I asked him to take me on as a student in his group. I was to come back the following Thursday to learn of his decision. That Thursday I entered his office with some trepidation. To my relief, he said “Yes, you can be my student.” Then he added, “Now you have two things you must do. The first is to find your research problem and the second is to solve it. Good day!”
In fact, Zener proved to be helpful. First, he gave me a Research Assistant position measuring the internal friction of iron wires doped with carbon or nitrogen. Second, once a week he had a bag lunch with his students. One of them was to give a lecture on some topic that he assigned. After my first lecture, he called me to his office and asked whether I had found a research problem in the topic assigned. So, this was his game! In my next assignment I found a topic that proved too hard for me to solve. In my third round, the problem I found was too easy. Finally, in my fourth round I found one that would work for me. I would calculate how the interaction of the Fermi surface with the Brillouin-zone boundaries of non-cubic metal alloys would influence or change their structure. In momentum space, the position of the Fermi surface of a metal depends on the electron density in the conduction band, and the Brillouin zone is determined by the translational symmetry of the periodic potential in which the electrons move.
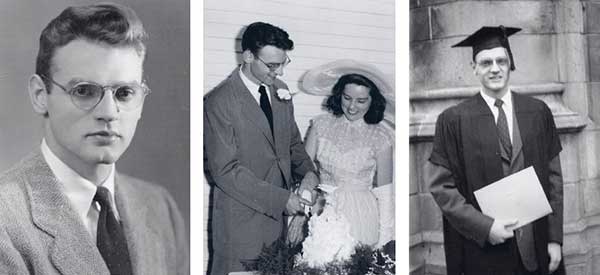
Figure 7. Left: Myself as a graduate student. Middle: Irene and I on our wedding day, June 1951. Right: My graduation from the University of Chicago, 1951.
While I was engaged with this problem, Zener took a job as Director of the Westinghouse Research Laboratory in East Pittsburgh, PA. He invited his students to join him. My position there as a Research Engineer my final year enabled me to get married to Irene Wiseman Goodenough the Spring before we moved to Pittsburgh. When I was writing up my dissertation, Zener informed me that my employment at Westinghouse would be terminated; I was to start looking for another job.
The week before my final defense back in Chicago, I went to the American Physical Society meeting in Washington D.C. to present my work and to look for a job. After my 10-minute talk, an old man in the front row stood up and said, “That’s fine, young man, but you do not have the correct Brillouin zone for the hexagonal-close-packed structure!” The old man was Brillouin. The Head of the Chicago Physics Department was in the audience and witnessed the embarrassed silence after Brillouin had spoken. I went back to my hotel room demolished! Any Ph.D. defense the following week would be lost, I thought. However, that weekend I was able to show that although Brillouin was mathematically correct, he was physically wrong. There is no energy discontinuity across the zone face that I had omitted. The zone that I had used was the one that was physically meaningful. When I defended my thesis in Chicago the next week, another professor challenged me. He had a student working on the same problem from a different point of view. This time I was able to better the challenge, and the confrontation had pleased the examiners. I was finally awarded the Ph.D. degree!
Lincoln Laboratory years
When I received my Ph.D., three options were offered to me: (1) to be an Assistant Professor in the Physics Department of the University of Pennsylvania, (2) to be a Research Engineer at the MIT Lincoln Laboratory, and (3) to be a Research Fellow at Harvard University. The position at the Massachusetts Institute of Technology seemed to be the best match for me, and I went to Boston with an inner assurance.
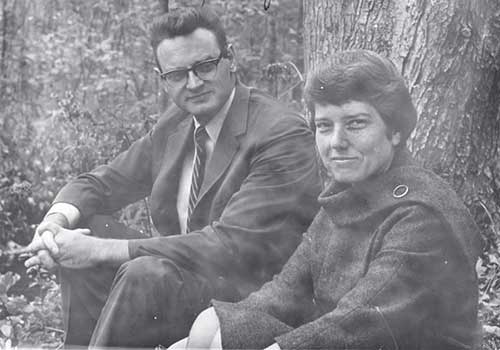
Figure 8. Irene and I in New Hampshire.
The MIT Lincoln Laboratory was supported by the Air Force to create a defense against aircraft; the ballistic missile was not yet a threat. The defense system brought together the radar installations developed at MIT during World War II, communications, and the digital computer. In 1952, the digital computer ran on vacuum tubes and filled the space of a large dance hall; but it had no memory. Jay Forrester of the Electrical Engineering Department of MIT had invented the concept of a random-access memory (RAM) storing binary numbers, 0 and 1. His memory used as the memory element a ferromagnetic transition-metal alloy with a square B-H hysteresis loop formed by rolling alloy tapes into thin sheets. Frustrated by the inability to switch the magnetization direction fast enough, Forrester had concluded that his problem was due to eddy currents in the metallic alloys. Since the entire project depended on a faster RAM memory of the digital computer, he decided to investigate the possibility of developing a square hysteresis loop in a ferrimagnetic oxide that was an insulator. Ferrimagnetic oxides had been developed secretly in France and Holland during World War II. I was assigned to a small group to develop the square hysteresis loop in a ceramic that cannot be rolled. The magneticians of the day assumed it would not be possible, but the group of ceramists and electrical engineers that I joined were empirically synthesizing and testing ferrimagnetic spinels that showed some promise; the oxospinels contained Mg, Mn, and Fe and were supplied by a small ceramics company.
Within three years, systematic empiricism and quality control enabled the experimentalists of our group to develop a recipe for reproducible fabrication of polycrystalline ceramic cores with the needed squareness of their M-H loop. This success involved optimizing the composition and specifying accurately the firing time at specified temperatures as well as the cooling rate for some unknown atomic-ordering process during synthesis. My contribution was, first, to identify the factors that controlled the shape of the M-H hysteresis loop and to show that the lower value of M in the ferrimagnetic oxides compared to the ferromagnetic alloys alleviated the requirement of aligned crystallographic axes between grains. Next, I showed that the switching speed was controlled by an intrinsic damping factor, not eddy currents, and by the magnitude of the driving field H, which was limited by the application to less than twice the critical field Hc at which the magnetization is reversed. We were fortunate to have a larger Hc in the oxides than in the rolled alloys. Although I had not yet identified the defect that triggered nucleation at Hc of a domain of reverse magnetization that would also grow at Hc to switch the total direction of M, I had shown that the atomic-ordering process occurring at the annealing temperature was associated with a critical concentration of manganese in the oxide. I had also recognized that a distortion from cubic to tetragonal symmetry occurring above this critical concentration of manganese was due to a cooperative orbital ordering at the manganese. This recognition introduced a fundamental new insight into a factor that determines crystal structure. It is now referred to as a cooperative Jahn-Teller effect since Jahn, as a student of Teller, had years before shown that where an isolated molecule has an orbital degeneracy in a state of high symmetry, the molecule is made more stable by a deformation to lower symmetry that removes the degeneracy. It was only some years later that I was able to show that the critical atomic order that we were controlling was a chemical inhomogeneity induced by a dynamic site distortion that cost less elastic energy in the crystal if it occurred cooperatively within manganese-rich regions. Nevertheless, I was able to predict that ferrospinels containing a critical concentration of Cu2+ ions would also yield the desired square M-H loop. I had also been able to apply the insight of a cooperative orbital ordering to articulate chemical rules for the sign, parallel or antiparallel, of the interactions between atomic magnetic moments. These rules are now known as the Goodenough-Kanamori rules; Kanamori subsequently provided a mathematical formalism justifying these rules.
One Friday afternoon after we had delivered the fast RAM with a ferromagnetic spinel memory element, Jay Forrester summoned the group to his office. I thought he might give us a raise. Instead, he thanked us for solving his problem and asked, “Now that you have worked yourselves out of a job, what are you planning to do?” The response of half of the group was to take their know-how to industry. My response was to spend the weekend thinking what we should do next. I came up with the idea of a magnetic-film memory in which all the individual atomic moments would switch simultaneously rather than sequentially. On paper it promised to increase the switching speed one-thousand-fold. However, magnetic cores could be made smaller and realization of reliable switching of films meant slowing of their switching time. Since the film technology was more demanding, the eventual difference in switching speeds was not great enough for a move to magnetic films except for a few niche operations. With the advent of fast transistors that could be miniaturized, the Whirlwind Digital Computer and its magnetic memory became obsolete, but this technology was a critical step in the evolution to today’s supercomputers and laptops.
With the exodus of half of the group and its leader, I was put in charge of the remnant and charged with realizing the magnetic-film memory and rebuilding the ceramic facility. After two years, I gave the magnetic-film project to Donald Smith who asked for it and devoted my time to devising experiments with transition-metal compounds, mostly oxides, that would reveal how competing interactions between atomic moments would give unexpected and/or complex magnetic order; I also investigated the role of cooperative orbital ordering in determining not only magnetic order, but also magnetostrictive phenomena that could be used in devices. This work resulted in my first book, published in 1961, “Magnetism and the Chemical Bond”. At the same time, I realized that the transition-metal oxides and sulfides also permitted a systematic study of the transition from the localized-electron behavior responsible for atomic magnetic moments to itinerant-electron behavior as the strength of the interatomic interactions between atomic magnetic moments increases beyond a critical strength. Intraatomic interactions localize the electrons to an atomic site; interatomic interactions delocalize them. Itinerant electrons belong equally to all the like atoms of a periodic array, which allows metallic conductivity and suppression of any spontaneous magnetism. Studies of phenomena at this cross-over led to a long review, “Metallic Oxides”, published in 1971; it was translated in French into a book, “Les oxydes des métaux de transition” published in 1973. Clearly, the move to Lincoln Laboratory had been a good match; it had allowed me to find my own scientific voice and to contribute at a critical point to the development of the digital computer, a revolutionizing technology for a growing, diverse global population.
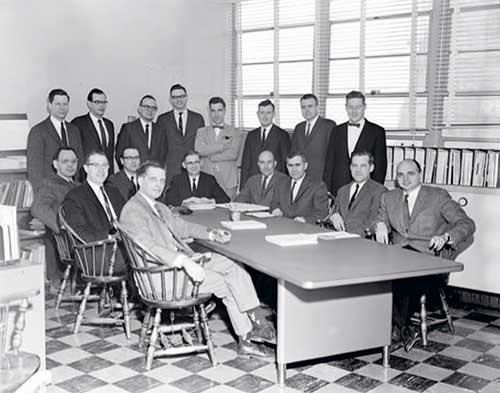
Figure 9. Myself (the fourth from left in the back standing) at a group meeting of Division 8 (Solid State Division back then) in Lincoln Laboratory in January 1964. (Reprinted with permission. Courtesy of MIT Lincoln Laboratory, Lexington, Massachusetts).
In the mid-1960s, I was moved with the ceramics and magnetic measurement part of my group to the Solid State Division where I was also to have charge of the chemists investigating the growth of single crystals and the semiconductive materials finding application in the blossoming fields of microelectronics, photovoltaics, lasers, and solid-state lighting. A high-pressure facility was also available in that group; it was a tool wellsuited for my studies of the transition-metal oxides. I transferred to my new group a vibrating-coil magnetometer we had built for the purpose of making magnetic measurements under pressure. Working with John Longo and James Kafalas was very productive. High-pressure synthesis and the ability to make magnetic measurements under pressure proved fruitful, but I was forced to suspend these studies in 1970 until I moved to Texas in 1986.
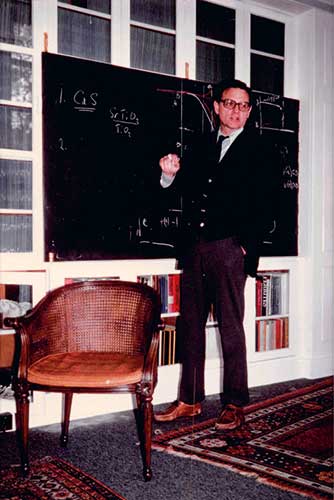
Figure 10. At my home office in Boston.
An amendment to a bill of Congress in about 1970 forbade research in a government Laboratory that was not targeted towards a specific application, and I was ordered to terminate my fundamental studies. At the time, I had just started a study of the copper-oxide system in which Bednorz and Müller made the discovery in 1986 of high-temperature superconductivity that won them the Nobel Prize in Physics; but I was not looking for superconductivity in 1970. However, I had done enough work on the transition from localized to itinerant electronic behavior to know that lattice instabilities are found at this crossover. This knowledge enabled me to identify the critical role of these instabilities not only in the surprising phenomenon of high-temperature superconductivity, but also in that of the colossal magnetoresistance discovered later in the manganese oxides. However, this insight was resisted by the orthodox theoretical physics community for nearly 20 years.
At Lincoln Laboratory in 1970, I turned my attention to the problem of renewable energy and energy conservation. It was obvious already in 1970 that our dependence on foreign oil was making the country as vulnerable as the threat of ballistic missiles from Russia. Solar energy was an obvious renewable source to be harnessed; our profligate use of energy made conservation an obvious target also. Since solar energy is variable in time and location, it was also obvious that we needed to find a way to store the solar energy that is converted into electricity. The best place to store electrical energy is to convert it to transportable chemical energy. Two clean routes to storing electrical energy in chemicals are electrolysis, as in the electrolysis of water to produce hydrogen, and in the anode of a rechargeable battery. Both options interested me. For improved efficiency of a power plant, I proposed use of the exhaust heat in a solid oxide fuel cell. Although we had, at Lincoln Laboratory, the facilities and interested scientists and engineers to tackle research and development targeting these applications, we were told that we were an Air Force laboratory, and that energy was to be the domain of the National Energy Laboratories and of Industry. Politics, not potential productivity, is the bull in the china shop of science administration. It was a discouraging moment, and I realized it was time for me to leave Lincoln Laboratory.
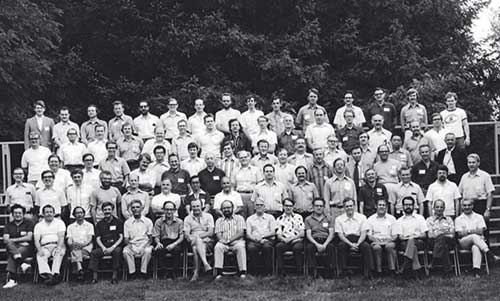
Figure 11. Gordon Research Conference, New Hampton School, August 1974. I am the third person from right on the back row.
Tenured years: Oxford
During the late 1970s and early 1980s, I continued my career as head of the Inorganic Chemistry Laboratory at the University of Oxford. In 1976, Oxford had four chemistry laboratories, each headed by a Class A Professor: Organic, Physical, Theoretical, and Inorganic.
The Oxford educational system provided me a relatively smooth transition from a research laboratory to an academic post. Most of the teaching is done by the Dons in the Colleges; lectures are a supplement. The Dons act as coaches to self-teaching in preparation for the big final examination at the end of the third year. They also act as admissions officers to their college and compete with the other colleges for best scores in the finals. Laboratory instruction, lectures, and research are carried out in the Chemistry Laboratory. Competition for scholarships for D. Phil students between the three large Chemistry Laboratories − Organic, Physical, and Inorganic − sometimes required diplomacy between the professors. Fortunately, good applicants to Inorganic Chemistry always put me in a favorable position.
For my research program, I initially selected two primary targets; the direct methanol-air fuel cell and the photoelectrolysis of water. The former requires for its electrolyte a solid proton (H+-ion) conductor that is an electronic insulator and can operate near 300°C or an anode that is catalytically active for the oxidation of methanol (CH3OH) below 80° C and chemically stable in an acidic solution. I soon found that good proton conductivity in a solid electrolyte only occurs where the solid is wet, which means operating below 80°C. Our unsuccessful search for a sufficiently active and chemically stable anode for a direct methanol-air fuel cell introduced me to the field of electrochemistry. Our attempt to realize a practical electrode for photoelectrolysis also involved electrochemistry. I had hoped to be able to use most of the spectrum of visible light by using a filled as well as a nearly empty d-electron band of a transition-metal oxide. However, the filled d-electron band proved to be too narrow for this strategy to be practical. The alternative was to attach a dye to the surface of the oxide. This exercise provided a good D. Phil. thesis, but it did not give a practical solution. I realized it would probably be better to separate the steps in the process by coupling a photovoltaic cell to an electrolysis cell in order to store solar energy as chemical energy in hydrogen gas, a portable fuel. More successful was my effort to identify a suitable cathode material for a lithium battery, an effort that has done much to bring together the solid-state chemist and the electrochemist.
The most mobile working ion in a rechargeable battery is the H+ ion. But these protons are only mobile in an aqueous acidic or alkaline electrolyte. To avoid electrolysis of the water, the single cell of an aqueous-electrolyte rechargeable battery is restricted to a voltage less than 1.5 V if the battery is to have a long shelf life. This restriction limits the energy density of a rechargeable battery, which is why the advent of the cellphone and the laptop computer had to await the arrival of the lithium rechargeable battery. The working Li+ ion of a lithium battery is mobile in a nonaqueous electrolyte, which permits single-cell voltages over 4 V. However, realization of a competitive rechargeable lithium battery requires identification of electrode materials into/from which Lithium can be inserted/extracted reversibly over a large solid-solution range. Moreover, the active redox couples of the insertion-compound electrodes must have energies matched to the allowable energy of the electrolyte if larger voltages are to be achieved.
Before I left Lincoln Laboratory, I had been asked to monitor the Na-S battery project at the Ford Motor Co. This assignment introduced me to electrochemistry and the problem of designing fast cation conduction in a solid electrolyte. With Henry Hong, a crystallographer and chemist, I explored framework structures for a 3D Na+ conductor as against the 2D Na+ conduction in β-alumina. We came up with the Na1+3xZr2(SixP2-xO4)3 framework structure that was called NASICON (NA SuperIonic CONductor) by colleagues after I had left for Oxford. This structure is now being explored further for cathodes and solid electrolytes of sodium rechargeable batteries.
In about 1974, Brian Steele of Imperial College, London, was aware of the Rouxel and Schöllhorn work on the chemistry of reversible Li+ insertion into layered MS2 sulfides and suggested at a conference the use of TiS2 as the cathode of a Lithium rechargeable battery. Primary Lithium batteries using a flammable organic liquid carbonate electrolyte with an ethylene-carbonate additive to passivate the Lithium anode from reducing the electrolyte had been marketed. M. Stanley Whittingham was a postdoc at Stanford with Bob Huggins and Fred Gamble was a physics student there with Ted Geballe studying intercalated TiS2 as a 2D superconductor. They were hired by the Exxon Mobil Corporation to develop a commercial Li/TiS2 rechargeable battery, and in 1976, Whittingham reported fast, reversible Li+ into TiS2 in a rechargeable Li/TiS2 cell. However, on charge, the lithium anode formed whiskers (dendrites) that grew across the electrolyte on repeated charges to cause an internal short-circuit that ignited the flammable organic-liquid electrolyte. This effort was, therefore, terminated in the U.S. However, the concept of a reversible intercalation of Li+ into layered compounds was established, and several laboratories were exploring Li+ insertion between the layers of graphitic carbon. At the SONY Corp. of Japan, they were planning to use lithiated graphite as the anode with a TiS2 cathode.
In 1978, an undergraduate thesis at Oxford on the structure of the LiMO2 oxides reminded me of work I had done with Donald Wickham in the 1950s on LixNi2-xO2. The MO2 oxides are not layered as is TiS2; the electrostatic repulsive energy between the O2- ions of MO2 sheets is larger than the dipole-dipole Van der Waals binding energy. However, layered LiMO2 is stabilized by the Li+ ions between the MO2, sheets, and the ions are well-ordered provided the sizes of the Li+ and M3+ ions are sufficiently different from one another. I decided to investigate how much lithium can be extracted reversibly from a well-ordered LiMO2 layered oxide. Since I wanted an M4+/M3+ redox couple that had an energy well below the Li+/Li0 couple of a metallic Lithium anode, I chose to study chromium, cobalt, and nickel for the M atom. An experimental physicist, Koichi Mizushima, had just come from the University of Tokyo to work with me at Oxford. I teamed him with my chemist post-doctoral assistant, Phillip Wiseman, to work on this investigation. We found that over half of the lithium could be removed reversibly with cobalt or nickel as the M atom; each of these Li1- xMO2/Li half-cells gave an output voltage near 4V.
No battery company in England, Europe, or the U.S. was interested in licensing a patent for these cathode materials; they could not imagine starting with a discharged cathode. The University of Oxford was not interested at that time in the intellectual property of its academics. As I was working with scientists of the AERE Laboratory in Harwell, a town near Oxford, to obtain joint funding for battery research from the European Economic Community (EEC), I arranged for them to apply for a patent on the understanding that, once they had retrieved their filing expenses, my two colleagues and I would share any revenue. On the day of signing, I was told that the AERE Harwell lawyers would not proceed unless we signed all our rights away. Not knowing either the full potential of our invention or any other option, we signed our rights away. Meanwhile, others were exploring the chemistry of Li insertion into layered compounds. In Switzerland, Rachid Yazami showed that reversible Li insertion/extraction into graphite occurs at only 0.2 eV below the electrochemical potential of metallic lithium without dendrite formation. In Japan, Akiro Yoshino of the Asahi Kasei Corp. then realized that he could assemble a discharged rechargeable battery using graphite as the anode and my LiCoO2 as the cathode. Scientists at the SONY Corporation commercialized this Li-ion battery to market the first cell telephone that launched the wireless revolution. AERE Harwell received many millions of pounds; we received nothing. I was disappointed that not even a contribution to St. Catherine’s College was forthcoming. However, the joy of having helped to enable a technology that has transformed for the better so many lives is reward enough.
Cobalt is expensive and toxic. In 1981, Michael Thackeray came from South Africa to work with me. He had been inserting lithium into magnetite, Fe3O4, the original ferrospinel used by the Greeks for navigation. He wanted to develop a less expensive cathode than Li1-xCoO2. Bruno Scrosati of Rome had reported a similar experiment in a seminar I had attended two weeks before Thackeray’s arrival. I was skeptical of this report as I knew that the spinel structure cannot tolerate excess cations. Therefore, I asked Thackeray, when he arrived, to repeat his experiment in my laboratory. When he confirmed the insertion of lithium into magnetite, I realized that the insertion of lithium must be displacing the tetrahedral-site iron to the empty octahedral sites of the structure to form an ordered rock-salt phase. This insight made me realize that the spinel octahedral-site [M2]O4 array represented a three-dimensional framework into/from which lithium could be inserted/extracted reversibly. Therefore, I told Thackeray to insert lithium into the manganese spinel Li[Mn2]O4.
The Li1+x[Mn2]O4/Li half-cell gave a flat 3 V open-circuit voltage. Thackeray would later extract lithium from the tetrahedral sites; the Li1+x[Mn2]O4/Li half-cell gave a 4 V open-circuit voltage. A sharp shift of 1eV in the energy of the Mn4+/Mn3+ couple occurs where the Li+ ions change their occupancy cooperatively from all-tetrahedral to all-octahedral sites. I gave Thackeray the patent rights to the spinel framework, but that patent was changed in South Africa to cover only the 4-V range of Lix[Mn2]O4 (0<x <1). Donald Murphy of the Bell Telephone Laboratory had independently prepared the spinel framework [Ti2]S4 by extracting copper chemically from Cu[Ti2]S4. In the sulfospinel framework, lithium enters only octahedral sites and the [Ti2]S4/Li half-cell gives a voltage identical to that of the original TiS2/Li half-cell. In LixTiS2, lithium also occupies only octahedral sites. Chemical instability on cycling Lix[Mn2]O4 over the 4-V solid-solution range 0< x <1 prevented its commercialization as the cathode of a lithium battery. However, addition of some nickel and lithium to the [Mn2]O4 framework has provided chemical stability at the expense of discharge capacity. NISSAN has used this stabilized cathode material in their initial hybrid car.
At Oxford, in addition to the cathode materials for a Li-ion battery, I learned about oxide surface reactions with the medium in which they existed, including the zeta potential in aqueous solutions and Li+ attraction to an oxide surface in a non-oxide solid to create Li+ vacancies for Li+ conduction in the non-oxide solid medium. With the realization of structural reconstructions at an oxide surface, I understood how it frustrated study of heterogeneous catalysis by oxides. Therefore, I investigated the partial oxidation of acrolein to methacrolein on a Keggin 12-molybdophosphate of known surface structure to determine the role of a stable reduced molybdenum Mov displaced from an oxygen vacancy in the catalytic process, a possibility occurring to me from my earlier studies of solid molybdenum-oxide chemistries.
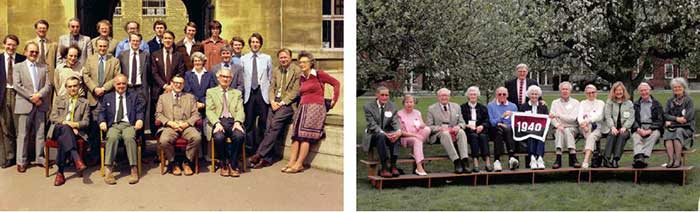
Figure 12. Left: Me (front row, second from right) with my colleagues at Oxford University, 1982. Right: Reunion of Groton School of the class of 1940 and their wives.
At Lincoln Laboratory I had initiated work to strengthen interactions between the solid-state chemist and solid-state physicist; at Oxford, I brought together chemistry and electrochemistry to stimulate both communities. It was for work fostering these interactions that I was to be awarded the Japan Prize in 2002 and given the highest awards of the Material Research Society, the Electrochemical Society, and the 3M Society.
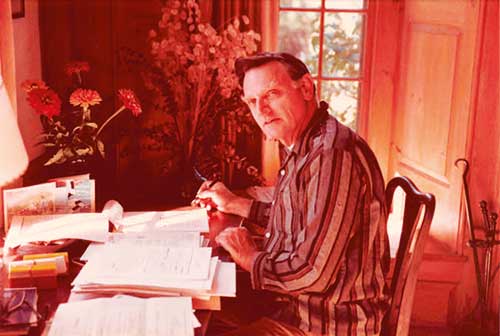
Figure 13. Working at my home office in New Hampshire.
Austin years
In 1986, the politics in the laboratory at Oxford anticipating my retirement had begun, so I accepted a call to take the Virginia H. Cockrell Centennial Chair of Engineering at the University of Texas at Austin. Retirement before age 67, was no longer required in the U.S. which has enabled me to keep working for another 32 years in Texas as a Professor of Materials Science and Engineering.
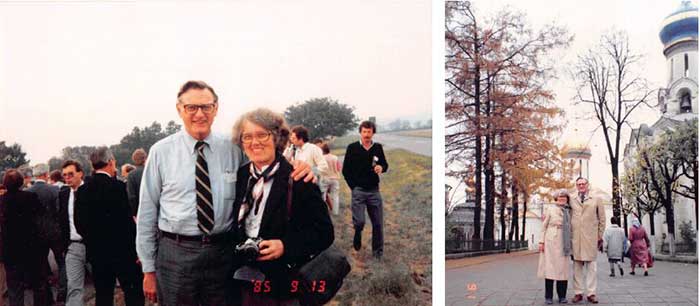
Figure 14. Left: Irene and I in Japan (1985). Right: Irene and I in Russia (1991).
In 1986, Bednorz and Mueller of IBM Zurich reported their discovery of high-temperature superconductivity below 40 K in a copper oxide while exploring whether a dynamic Jahn-Teller electron-lattice interaction at octahedral Cu ions might provide a needed electron-lattice interaction for superconductivity. Their superconductive compound was shown in Japan to be La2-xSrxCuO4. While I was setting up a chemistry laboratory in Texas with the aid of a postdoc, Arumugam Manthiram whom I brought with me from Oxford, a superconductive transition at 90 K in YBa2Cu3O7-δ was announced. Hugo Steinfink, a crystallographer in our ME Department who had supervised Henry Hong and had brought me to Texas, determined the structure of the 90 K superconductor the day before the structure was also announced at the ”Woodstock of Physics” in New York. Subsequently, Arumugam Manthiram and I explored extensively the chemistry of the copper-oxide superconductors.
In 1987, a letter from the University of Jilin in China asked if I would take a physics student to do his Ph.D. thesis with me for graduation from the University of Jilin. The physics student is now Research Professor Jian-Shi Zhou in my group; interested in high-pressure studies of solids; he had followed the high-pressure work we did at the MIT Lincoln Laboratory. Since a discarded and broken copy of the Kafalas high-pressure cell resided in the laboratory of Hugo Steinfink, I accepted Jian-Shi Zhou, who was able to refurbish the Kefalas cell; and he has remained with me ever since. Over the last 30 years, Jian-Shi Zhou has built up a competitive high-pressure facility, a single-crystal furnace, the ability to synthesize under pressure to 26 GPa, and to measure structures as well as magnetic and transport properties under pressure and the thermal properties of his materials at ambient pressures.
At MIT, we had shown that SrRuO3 is a ferromagnetic metal and that the paramagnetic susceptibility of the Sr1-xCaxRuO3 system exhibits a change to a negative Weiss constant, but with no long-range magnetic order in CaRuO3. Much later, Rob Cava of Princeton University showed a peculiar transition in the paramagnetic susceptibility of Sr1-xCaxRuO3 near the Curie temperature of SrRuO3, and Jian-Shi Zhou pointed out that the Cava data represented formation of a Griffiths phase in which the magnetic ions are diluted by nonmagnetic ions, which means there is a segregation into RuIV with magnetic 4d electrons and RuIV with nonmagnetic 4d electrons localized by spin-orbit coupling. He further found itinerant-electron ferromagnetism in the high-pressure Sr1-xBaxRuO3 perovskites with an abrupt transition under pressure from ferromagnetism to Pauli paramagnetism in cubic BaRuO3. The structure-composition property relationships in the single-valent oxoperovskites have been shown to be rich as also have the mixed-valent manganese perovskites.
On arriving in Austin, I set up my chemistry laboratory for work on electrochemistry and ionic transport in solids with a view to continue work on energy-related materials. We finally had a chemical hood installed by Christmas! By that time, not only had the structure and composition of the original copper-oxide superconductor been identified; doping of the phase had led to the discovery of another copper-oxide phase that becomes superconductive at 90 K, well above the boiling point of liquid nitrogen! Announcement of this discovery in the New York Times created a stampede of crystallographers in Japan and the U.S. to be the first to determine the structure of this new phase. Hugo Steinfink solved the structure and was the first to announce it at a crystallographic meeting in Austin the day before the “Woodstock” of the American Physical Society Meeting in New York City where other groups also announced the solution. Steinfink’s contribution was overshadowed by the trumpets of the larger laboratories that had solved the structure independently.
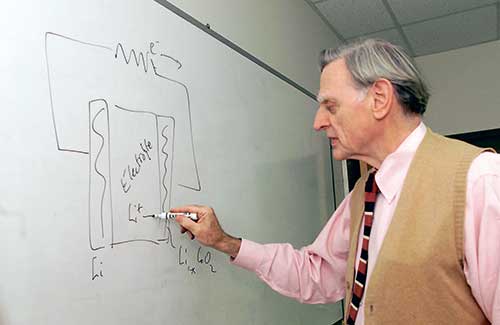
Figure 15. Drawing a diagram of a battery on the board in my office at the University of Texas in Austin, 2012.
Although I had not previously been interested in working on the superconductive phenomenon, this development in a transition-metal oxide captured my attention; Manthiram and I began to study the chemistry of these copper oxides. It became immediately evident to me that the extraordinary superconductivity in the copper oxides was occurring in a phase intermediate between an antiferromagnetic parent compound that was an insulator and a non-superconductive metallic phase. Superconductivity was appearing at a much higher temperature than predicted by the existing Bardeen-Cooper-Schrieffer theory and at a transition from localized to itinerant electronic behavior in a transition-metal ceramic, a transition that I had explored in these perovskite-related oxides in the 1960s. However, in this case the crossover was occurring in a mixed-valent system whereas I had been studying it in single-valent systems. I knew that lattice instabilities were always encountered at this crossover in single-valent systems, so I suspected that a dynamic segregation of localized and itinerant electrons was occurring in the mixed-valent copper oxides. This idea was aggressively dismissed by the leading solid-state theorists who were the opinion leaders for the majority of physicists; but when Zhou arrived, I persisted to explore this possibility with him. Manthiram became a professor in his own right and returned to the development of energy materials independently of me.
We had a first-rate high-pressure facility in Austin. Together, Zhou and I have explored the transition from localized to itinerant electronic behavior in other mixed-valent and single-valent transition-metal oxides with perovskite-related structures. We have clearly demonstrated a dynamic segregation into localized-electron and itinerant-electron domains in other mixed-valent systems as well as in the copper oxides.
The copper-oxide problem is complicated by the existence of two types of phase segregation, one a dynamic segregation into localized-electron and itinerant-electron domains and the other a static phase segregation of the superconductive phase from the antiferromagnetic parent phase on the one side and the metallic over doped phase on the other side. As the oxidation state of the superconductive CuO2 sheets of the copper oxides increases, the charge carriers introduced by the oxidation change their character. In the antiferromagnetic phase, isolated carriers occupy a volume of about 6 copper centers. Where the oxidation is too great for the charge carriers to remain isolated from one another by electrostatic coulomb forces, they condense below room temperature into spin-paired carriers in a volume of four copper centers or into multiple spin-paired carriers in itinerant-electron chains along the Cu-O-Cu bond axes. This phenomenon represents a dynamic phase segregation in which the charge carriers are mobile. A structural distortion may trap the carriers in static itinerant-electron stripes separated by ribbons of localized electrons. These static stripes have been observed by conventional neutron-diffraction experiments, but a dynamic phase segregation requires a faster experimental probe. A pulsed neutron-diffraction experiment coupled with a pair-distribution-function analysis of the data is such a probe. This technique was developed by Takeshi Egami while he was at the University of Pennsylvania. His preliminary data show evidence of the predicted phase separation, but the complexity associated with isolated charge-carrier pairs coexisting with chain segments has made difficult a definite statement about the nature of the dynamic normal state in the superconductive phase. We have established the existence at room temperature of isolated charge carriers with a volume of 5 to 6 copper centers in the underdoped phase; in the metallic overdoped phase the localized electrons appear only as fluctuations. How the paired charge carriers become long-range ordered to give superconductivity is still to be resolved. I have pointed out that this can be done by coupling the domains of paired electrons to cooperative lattice vibrations (phonons) to give vibronic charge carriers.
In my chemistry laboratory, we also continued to develop materials for the lithium rechargeable battery and the solid oxide fuel cell. I assigned to my engineering student, Akshaya Padhi, and my post-doctoral fellow, Nanjunda Swami, the task to explore the relative energies of transition-metal redox couples in the NASICON framework M2(XO4)3 that we had shown in Lincoln Laboratory supports fast transport of the Na+-ion guest species. Different transition-metal atoms M and polyanions (XO4) can be accommodated in the framework, with the charge of the framework being balanced by Li+-ion guests over the range 0< x <5 in LixM2(XO4)3. In this framework, the energy of the redox couples appear to be essentially insensitive to the location and number of Li+ ions in the interstitial space. I was also interested in knowing how those redox energies shifted on changing from (SO4)2- to (PO4)3- or (AsO4)3- polyanions.
During the course of this work, Padhi found that Lithium can be extracted reversibly from LiFePO4, which has the olivine structure. The LixFePO4/Li cell gives a constant 3.45 V open-circuit voltage over the range 0< x <1. Made as small particles, this cathode is capable of extremely fast rates of charge and discharge. The Hydro Quebec Corporation licensed the patent granted to the University of Texas; but the A123 company in Cambridge, Massachusetts, was the first to market the LiFePO4/C battery and to demonstrate its use in medium-power applications such as electric power tools and small electric vehicles.
Our work on the solid oxide fuel cell has involved the development of new oxide-ion electrolytes as well as new electrodes. Dr. Kevin Huang worked with me as a post-doctoral fellow on these problems before going to become a key player in the Siemens-Westinghouse development of a commercial hydrogen-fueled solid oxide fuel cell. After his departure, I investigated a novel class of anode materials that can operate on natural gas without becoming poisoned by sulfur impurities in the gas. The move from the internal combustion engine to batteries to power our automobiles would reduce distributed CO2 emissions responsible for global warming; the development of electrical energy storage is needed to make viable the substitution of solar, wind, and nuclear energy sources for the fossil fuels that emit CO2 on burning; the sequestering of CO2 and other pollutants emitted from coal-fired power can reduce CO2 emissions; and the introduction of more efficient energy distribution and use can reduce energy consumption. All represent urgent challenges confronting the scientific-engineering community today. The implementation of a serious national effort to meet these challenges, an effort initiated in the early 1970s, was stalled by special interests more concerned with profits than with our national vulnerability and the global environment.
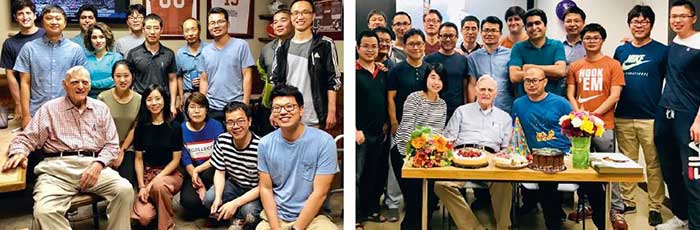
Figure 17. Left: With my research group, Austin, 2019. Right: My 96th birthday, July 2018.
In the end, I have had an extraordinary journey, but it is the many colleagues who have worked with me over the years that I wish to thank for making it extraordinary. They are the ones who have performed the experiments and each of them kept an open dialogue with the aim to teach me as much as I tried to teach them.
References
John B. Goodenough, Witness to Grace, PublishAmerica, 2018, ISBN: 1-60474-767-6.
This autobiography/biography was written at the time of the award and later published in the book series Les Prix Nobel/ Nobel Lectures/The Nobel Prizes. The information is sometimes updated with an addendum submitted by the Laureate.
John B. Goodenough died on 25 June 2023.
Nobel Prizes and laureates
Six prizes were awarded for achievements that have conferred the greatest benefit to humankind. The 12 laureates' work and discoveries range from proteins' structures and machine learning to fighting for a world free of nuclear weapons.
See them all presented here.