Elizabeth H. Blackburn
Biographical
Childhood
I was born in the small city of Hobart in Tasmania, Australia, in 1948. My parents were family physicians. My grandfather and great grandfather on my mother’s side were geologists. My great-grandfather on my father’s side, before coming to Australia as a minister of the Church of England, had lived for some time in Hawaii, where he had collected Coleoptera (beetles). He continued his collecting in Australia, eventually selling his collection to the British Museum of Natural History. My uncle and aunt (my father’s sister and my mother’s brother) were also both family physicians, who moved to England, married there and permanently settled there to practice medicine and raise their families.
I was the second child of eventually seven siblings. I spent my first 4 years living in the tiny town of Snug, by the sea near Hobart. Curious about animals, I would pick up ants in our backyard and jellyfish on the beach. Then my family moved to Launceston, a town in northern Tasmania. Our first house, at 120 Abbott Street, was a one-storied, verandahed house of typical Australian suburban architecture. I started kindergarten at a girls’ school, Broadland House Girls Grammar School in Launceston (Figure 1).
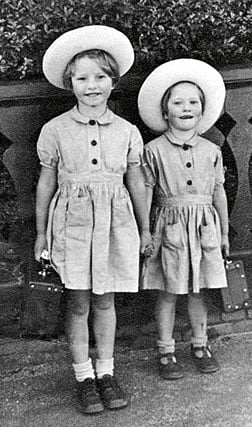
Figure 1. Elizabeth Blackburn (right) and her sister Katherine ready for Elizabeth’s first day at school in Launceston, Tasmania. Circa 1953.
I kept tadpoles in rapidly-smelly-becoming glass jars in a back living room at home. When I was a preteen we moved to a larger house called Elphin House, which had a good-sized garden (Figure 2). Over the years we had many pets: at one stage I enumerated the family menagerie of the moment as consisting of budgerigars and canaries in an aviary in one corner of the garden, goldfish in a garden pond, chickens and pullets (for eggs and the occasional roast fowl) in a hen coop and henhouse, rabbits and guinea pigs in cages, and cats and a dog, who lived all over the house and garden. I was fond of all these animals, and of animals and nature in general.
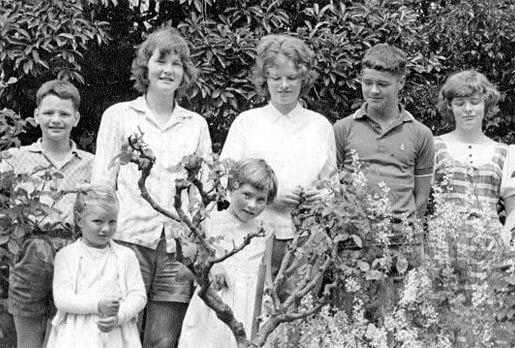
Figure 2. All seven Blackburn family siblings in the garden at their home, 3 Olive Street, Launceston, Tasmania. From left to right, back row: Andrew, Elizabeth, Katherine, John, Barbara; front row: Caroline, Margaret. Circa 1965.
Perhaps arising from a fascination with animals, biology seemed the most interesting of sciences to me as a child. I was captivated by both the visual impact of science through science books written for young people, and an idea of the romance and nobility of the scientific quest. This latter was especially engendered by the biography of Marie Curie, written by her daughter, which I read and reread as a child. By the time I was in my late teens it was clear to me that I wanted to do science. I was educated at Broadland House Girls Grammar School, and received a generally excellent education. However, physics was not offered, so I took physics classes offered in the evenings at the local public high school. Latin and Greek were not taught at my girls’ school either, a gap in my education that I rather regret later in life. But my school did provide an excellent piano teacher, Helen Roxburgh, by whom I was taught all throughout my school years in Launceston. I loved playing the piano, and even at one time wistfully hoped that I might become a musician. Fortunately I was also quite realistic about this, because I recognized that I was competent rather than greatly talented at piano playing, so I went in the direction of science.
My family moved, after some family disruptions, to the city of Melbourne, Australia, in time for me to complete my last year of high school at University High School. There I gained the confidence that I needed to apply for the undergraduate science degree at the University of Melbourne.
University Education
I chose biochemistry as my major and graduated after 4 years with an Honours degree in Biochemistry. During that time, I had come to love biochemistry research, although I was just getting my feet wet in laboratory research.
The Chair of the Biochemistry Department, Frank Hird, then offered me a position as a Master’s student in his research laboratory, where they investigated the biochemistry of amino acid metabolism. My undergraduate Honours thesis research advisors, Theo Dopheide and the late Barrie Davidson, had advised and encouraged me to do my Ph.D. abroad. Barrie in particular had urged me to consider going to the MRC Laboratory of Molecular Biology (LMB) in Cambridge, England, where he had done postdoctoral research. But in order to be accepted as a Cambridge Ph.D. student in biology, those from outside Britain were required to have done a year of research. The Master’s degree with Frank Hird, studying the metabolism of glutamine in the rat liver, would constitute this required year. Frank Hird taught his laboratory group members the joy and aesthetics of research. He said he thought each experiment should have the beauty and simplicity of a Mozart sonata. His laboratory group, dominated by his strong personality, was cohesive and we would sometimes drive to the hilly areas outside Melbourne, all piled into his car, Mozart playing loudly on the car radio, to have an outdoor lunch picnic among trees and wildflowers. While I was still in Frank Hird’s lab, Fred Sanger visited Melbourne. Frank Hird had done research in England on amino acids with Fred Sanger shortly after the Second World War, providing an introduction to Fred in which Frank encouraged me to tell Fred of my hope to study for my Ph.D. in Cambridge at the LMB. It was arranged that I would join Fred’s lab in the LMB and I was admitted as a Cambridge Ph.D. student.
The adventure of setting off to England, away from home and family, was a huge step, but I felt ready. My aunt and uncle and their family in Cambridge, who lived close to the LMB, became my anchor of a family away from home. I loved the LMB, the science being done there, the atmosphere of being at the epicenter of molecular biology, the intensity of the scientists and the constant discussions about science. It was a world of complete immersion. For my Ph.D. thesis research, I carried out sequencing of regions of bacteriophage phiX 174, a small single stranded DNA bacteriophage. I transcribed fragments of the phage DNA into RNA and then used the methods that Fred had pioneered for piecing together RNA sequences. We combined the sequences derived by this method with the DNA sequencing that had been done by John Sedat and Ed Ziff and Francis Galibert, members of Fred’s lab. All the sequences jibed. The first sequence of a 48 nucleotide fragment of this tiny bacteriophage DNA genome was a great excitement. I took it to show to my mathematically-talented Cambridge cousin, who was then about 12 years old, to see if any patterns emerged to his mathematically-inclined eye. He pointed out the repeats, but it was premature to think of analyzing DNA sequence patterns!
To the United States
The world of discovering DNA sequences was opening up and I was entranced by its possibilities. I had planned to do a postdoctoral fellowship, beginning in 1975, with Howard Goodman and his close associate Herb Boyer of UCSF, a mutual decision made after an interview–cum-conversation Herb and I had walking in the garden of a monastery in Belgium at which we were attending a scientific conference, I still as a graduate student. But then love intervened: John Sedat and I decided to marry, and as John was going to Yale, I decided to see if I could change my postdoctoral research plans (for which I had obtained an Anna Fuller Fellowship to work at UCSF) to a laboratory at Yale. Howard Goodman wrote me a kind and understanding letter upon my letting him know the reasons for my change of plan, and I began inquiries into possibilities of a laboratory for my postdoctoral training at Yale.
Thus it was that love brought me to a most fortunate and influential choice: Joe Gall’s lab at Yale. After a few hiccups engendered by misplaced international mail and other factors, at the beginning of 1975 Joe accepted me as a postdoctoral fellow in his lab, to which I was allowed to transfer my Anna Fuller Fellowship. I immediately began to work on finding ways to accomplish the sequencing of the DNA found at the terminal regions of the abundant, short, linear ribosomal gene-carrying “minichromosomes” that Joe and his colleagues, in parallel with Jan Engberg of Denmark, had discovered in the somatic nucleus of the ciliated protozoan Tetrahymena thermophila (which was at that time called Tetrahymena pyriformis, shortly thereafter to be renamed Tetrahymena thermophila).
To the University of California
After finishing my postdoctoral training in Joe Gall’s lab at the end of 1977, John Sedat and I, having married in 1975, moved to San Francisco, California. There John had accepted a position as Assistant Professor at the University of California San Francisco (UCSF). I had applied for several positions as an Assistant Professor in a variety of Universities and had been rejected from many of them, a discouraging experience. I had applied for such a position in the Department of Molecular Biology at the University of California Berkeley, but had not yet heard whether I was in the running for it. In the meantime, UCSF offered me a research track position and space in the Department of Biochemistry in the Genetics unit headed by Herb Boyer. My first NIH grant was the source of funding for my salary and research expenses. I had written this grant application with the encouragement of UCSF, in order to pursue my research on Tetrahymena telomeres and their associated proteins. This work grew out of that I had done in Joe Gall’s laboratory at Yale. My grant was funded by the NIH General Medicine Institute. Unsure of my chances at obtaining funding, I had sent the same grant application to the National Institutes of Health, the National Science Foundation and the American Cancer Society, hoping for funding from any one of these. Reflecting the more informal scientific habits of the basic sciences community in those days, some time later one of the grant reviewers told me that he had been so intrigued by my photograph of the autoradiogram, showing that telomeric DNA in Tetrahymena was mysteriously packaged as something other than nucleosomes, that he had kept the photograph.
Then UC Berkeley offered me an associate professor position in the Molecular Biology Department, which I immediately accepted. Once again, I transferred my funding from UCSF, this time to my own laboratory, at UC Berkeley.
Because research was, and still is, such a central part of my life, my autobiography would be incomplete without describing my research experiences. Thus, to convey a fuller flavor of them, here I describe some of the events of my early scientific research on the molecular nature of ends of chromosomes.
Early Work on the DNA at the Ends of Eukaryotic Chromosomes
Very soon after arriving in Joe Gall’s laboratory at Yale in early 1975, I started to apply methods for obtaining terminal DNA sequences to the Tetrahymena rDNA molecules. I had learned a collection of methods in Fred Sanger’s laboratory in Cambridge, England, where I had just completed my Ph. D. Eager to sequence the end regions of these minichromosomes, with Joe’s encouragement I set out right away early in 1975 to use end-labeling techniques on them. I incorporated 32P isotope-radiolabeled deoxynucleosides residues into the Tetrahymena rDNA molecules using commercially available DNA polymerases for in vitro DNA repair enzymatic reactions. The results were immediately promising. First, it became clear that the end regions of the rDNA were being selectively labeled by certain combinations of 32P isotope radiolabeled nucleoside triphosphate substrates. And by June 1975, I had become tremendously excited: I had obtained my first autoradiogram of the two-dimensional separation of the 32P labeled depurination products. A strong signal of a run of 4 cytosine (C) residues was apparent. Furthermore, each such C4 sequence was flanked by a purine residue (that is, an adenosine (A) or a guanosine (G) residue; this initial data did not show which). The way the depurination reaction worked was the following: Ken Burton, a New Zealander, had shown that a chemical reaction could be done that cleaves the DNA backbone on both sides of every purine nucleotide but leaves intact any runs of pyrimidine nucleotides (such as C residues) that are uninterrupted by purine nucleotides. This so-called depurination method, when applied to a complex sequence DNA like a whole bacteriophage genome, was further made useful by Vic Ling, when he was a postdoctoral fellow in Fred Sanger’s lab in Cambridge, England. Vic had shown that the resulting short pyrimidine tracts (mono-, di- tri-nucleotides, etc) would yield a pattern of products like a grid when a 2D fractionation method was used (see 2009 Nobel Lecture by Elizabeth Blackburn, in this volume). The most frequent products, on a random basis, of course are mono and dinucleotides, with the longer tri- and tetranucleotide tracts being less and less abundant on a random basis. Thus this strong C4 spot was interesting and informative.
It was also clear to me that the rDNA molecules were not simply lambda phage-like DNA. First, the terminal fragments were heterogeneous. In contrast, for any one type of lambdoid phage, in every viral particle the DNA molecule is just the same − a perfect carbon copy − as in every other viral particle. Second, on a per DNA molecule basis, much more incorporation of radiolabeled nucleotide precursor substrates occurred than expected if rDNA molecule ends were like those of the sticky DNA ends of lambda phages. It was also fortunate that the C4 repeat had such a regular nature and that it happened to have four Cs in a row. Even three C’s would have been striking, but harder to unravel. As it was, the C4 spot consistently stood out like a beacon through my repeated productions of 2D fractionations (“homochromograms” as we called them in Fred Sanger’s lab).
Next, I needed to validate independently that what I was radiolabeling in vitro validly reflected the rDNA sequence, and also try to get a closer estimate of the number of C4 runs per rDNA molecule. I therefore decided I needed to 32P isotope-label the rDNA in vivo. The vast majority of the 32P isotope (chemically in the form of inorganic phosphate ions) taken into the cells would be incorporated into other molecules, including the much more abundant cellular RNA, with very little ending up in DNA, and even less in the rDNA (in Tetrahymena, only a percent or so at most of the DNA is rDNA). Therefore, with some trepidation I asked Joe Gall for permission to order sufficient 32P phosphate to be added to the cell growth medium for labeling the rDNA. This meant handling 2 milliCuries at once, which Joe’s lab had not done before. But I knew that this was the only way currently available to get a sufficient amount of 32P into rDNA to detect the C4 spot in an autoradiogram, above background. Possibly with some trepidation on his side too, Joe agreed. I worked in the “hot room”, a room set aside for doing work with radioactive isotopes. On October 9, 1975 the 32P was shipped to Yale. My laboratory notebook from that time reads: “32P stored in refrig. until use – assayed for 10/14 . 3 pm 10/14. Zeroed [the cell culture] on 1% PPS medium blank … Added 2 mCi 32P as h3 PO4 in water in 1 ml from syringe.” For the next preparation I raised it to 5 milliCuries. Curious about this very radioactive departure from the more usual activities of the lab, my Gall labmates periodically looked in through the window set in the door of the hot room as I worked.
By October 22, 1975, I had the 32P rDNA purified. I triumphantly wrote down in my notebook that day my plans for this precious sample:
“1) Depurination
2) denaturing gel after EcoRi treatment
3) 1.4% agarose gel”
One by one, I inflicted various nucleases on the terminal region of the rDNA. I found it could be selectively radiolabeled using one triphosphate 32P labeled at a time. Then I carried out the battery of analyses possible at the time that would allow me to piece together the nucleotide sequence: I digested the radiolabeled end regions with Endonuclease IV nuclease or micrococcal nuclease, and performed depyrimidations and nucleotide nearestneighbor analyses, and spleen phosphodiesteriase digestions (Figure 3).
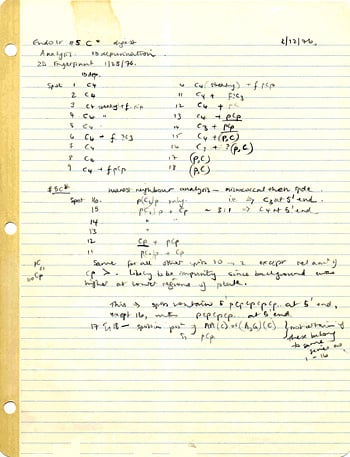
Figure 3. Lab Notes 02/12/1976.
Piecing together the rDNA end sequence was a matter of careful puzzle-solving. At an intermediate point, my notebooks of the time show that I had to consider two possibilities – CCCCAG and CCCCAA repeats (Figure 4). But it was apparent that there were a large number of repeated copies.
Figure 4. Lab Notes.
But by April 8, 1976, I was confident that the correct sequence was deduced, because the entry in my laboratory note-book page headed with that date reads:
“Sequence data from: | |||
1) Depurination | |||
2) Endo IV | – deps [my abbreviation for depurinations] | ||
– nearest neighbours | |||
3) partial micrococcal | |||
– deps | |||
– nearest neighbours | |||
partial spdes [my abbreviation for spleen phosphodiesterase digestions] | |||
4) Gel analysis of Endo IV digest of restriction fragments t ends of rDNA | |||
→ AACCCC repeated | |||
→ AACCCCAACCCCAACCCC) etc.” |
Another laboratory note-book page, dated August 17, 1976, shows I was already referring, in a routine way, to (CCCCAA)n sequence – by then in the course of experiments designed to see whether this same repeated sequence was also present in the other (much longer) chromosomal DNAs of Tetrahymena.
I put together a picture which tried to take into account all of my many observations. The deduced sequence consisted of a tandem array of CCCCAA repeats. One experiment done in 1979, radiolabeling the rDNA using just 32P-labeled dCTP, and unlabeled dAT P, and separating the products on a denaturing gel electrophoresis, showed this visually as a beautiful ladder of tiger stripes extending up the gel. The size of every band in this regular ladder was 6 bases more than the band below it! This strikingly characteristic visible pattern of bands presaged the pattern that would later become important for our discovery of telomerase enzyme activity, as described in this volume in the 2009 Nobel Lecture by my co-awardee, Carol Greider.
In the months following April 1976, much of my effort was also devoted to trying to understand the arrangement of the strand discontinuities along the tract of CCCAA repeat DNA. For this, I performed a great many experiments following the kinetics and specificity of radiolabeling the RNA end regions, using multiple different enzymes and protocols and analyses. This also was a matter of piecing things together – there was no template for me to work from as this was all uncharted territory.
In the late 1970s and early 1980s, I did a variety of radiolabeling experiments trying to divine the structure right at the termini of telomeres of both ciliated protozoans and yeast linear plasmids. I could put together a composite but still incomplete picture. Some of the features, I realize in retrospect, might be attributed to the terminal G strand sequence at the very ends of the telomeres assuming G-G paired or G-quartet structures. But other features are not so readily explainable. Why I was able to label the strands with DNA polymerase or a kinase to get the patterns of labeled strands and nucleotides I did has still not been completely fitted into a coherent view of the molecular structure of DNA ends. Various kinds of in vitro radio-labeling experiments had suggested that, in both Tetrahymena rDNA and the macronuclear DNAs of hypotrichous ciliates, there is a short overhang of the G-rich strand consisting of a few telomeric repeat sequences. Currently the view is that in mammalian telomeres there is a long protruding G-rich strand. Yet this does not take into account the clear evidence for the short C strand repeat oligonucleotides that I discovered can be readily melted off the telomeric DNA. This I found for both the Tetrahymena rDNA minichromosome molecules and linear plasmids purified from yeast. These tiny telomeric sequence oligonucleotides could be radiolabeled and clearly identified by two dimensional fractionations. However their significance is still unknown. To this day, aspects of the structure at the very terminal region of the telomeric DNA are enigmatic; the very ends of chromosomes remain as challenges.
Telomere Proteins: Earliest Attempts and Failures
In my early work, my molecular views of telomeres were first focused on the DNA ; not only because DNA was uppermost in my mind, but for several years DNA was also the only component of the telomeres that was identified. This was not for want of trying. I thought that DNA would not be the entire story of chromosome ends and, by extension from work emerging about chromatin in general in the 1970s, that it was likely that the telomeric DNA repeats tract would be packaged with proteins. The 1970s had seen great interest in chromatin, and the discovery of nucleosomes as the basic packaging unit of eukaryotic DNA. Telomeric sequences in Tetrahymena looked very intriguing to me in that regard, and as soon as I had identified the telomeric DNA I wanted to get my hands on whatever packaged it. Therefore, while still a postdoctoral fellow in Joe Gall’s laboratory, I performed micrococcal nuclease treatment on isolated Tetrahymena nuclei. I found that the CCCCAAn tracts of the telomeres were protected in chromatin as a heterogeneous class of DNA fragments very different from that expected for nucleosomal packaging.
Soon after moving from Joe Gall’s lab at Yale, while still temporarily at the University of California San Francisco (UCSF) in an independent research position (before I moved to the University of California Berkeley as an Assistant Professor), on March 1, 1978, I wrote to Joe Gall: “I am getting quite excited about getting a CCCCAAn-binding protein complex from the Tetrahymena macronuclei, so I’ve been busily making rDNA, the CCCCAAn probe, and macronuclear micrococcal nuclease digests. Results so far are that I’ve found a simple salt fractionation that enriches for CCCCAAn sequence plus putative protein(s). The plan at the moment is to purify this some more so I can get some structural characteristics of any such complex, i.e. S value, and some identification of protein(s) in terms of 1-D and 2-D gel eletrophoretic properties … The other aspect of course is to fish for something that will stick to a CCCCAAn column.”
Figure 5. Scanned page from grant application, 1977.
By 1980 I had done experiments to show that telomeric tracts of DNA in Tetrahymena were encapsulated in a protective sheath of protein that did not include nucleosomes. The vast majority of chromosomal DNA is packaged as nucleosomes: DNA-protein complexes. Each nucleosome is a flattened ball made up of histone proteins, around which the DNA is wrapped twice. The very basic (positively charged) histone proteins neutralize the negative charges of the phosphate chemical groups arrayed along the phosphodiester backbone of DNA and allow chromosomal DNA to become very closely packed and compactly folded in the nucleus. Nucleosomes in artificially stretched-out chromosomes are like beads on a string, although mostly in the nucleus they are closely packed into shorter thicker fibers. If one clips up chromatin using an enzyme, micrococcal nuclease, that cuts across the two strands of the linker DNA between neighboring nucleosomes, after getting rid of the histones, one can see that there are nucleosome-sized fragments of DNA left – a fragment of about 142 base-pairs is protected by the histone core of the nucleosome, once the DNA linkers have been trimmed away. This kind of nuclease clipping behavior is a hallmark of a nucleosome. In contrast to nucleosomal regions of chromosomes, special regions of DNA, for example promoters that must bind transcription initiation factors that control transcription, have proteins other than the histones on them. The telomeric repeat tract turned out to be such a non-nucleosomal region. We found that if we clipped up chromatin using an enzyme that cuts the linker between neighboring nucleosomes, it cut up the bulk of the DNA into nucleosome-sized pieces but left the telomeric DNA tract as a single protected chunk. The resulting complex of the telomeric DNA tract plus its bound cargo of protective proteins behaved very differently, by various tests, from standard nucleosomal chromatin, and therefore we concluded that it had no histones or nucleosomes.
By 1977, it was known from work of Rekosh et al. that adenovirus DNA has a covalently bonded terminal protein, presumably for viral genome replication. Thus, in 1979, Marsha Budarf, a postdoctoral fellow in my laboratory at UC Berkeley, began using used radioactive iodine procedures (the Bolton-Hunter reagent) to see if we could find any comparable protein at the ends of rDNA. Although Marsha found a covalently attached protein (that in hindsight may have been topoisomerase I) enriched toward the end of the rRNA transcribed region, it was not enriched in the terminal parts of the rDNA molecules. She was unable to detect any other covalently attached protein elsewhere on the rDNA. Any evidence for a protein on the bulk of the rDNA molecule ends, such as their behavior in gel electrophoresis and the appearance of the rDNA molecules under the electron microscope, was conspicuously lacking. This made me feel all the more confident that there was no covalently attached protein at the very ends of this minichoromosome. But what other proteins were at telomeres?
My lab was the first to try to identify these protective proteins. We used biochemical fractionations of Tetrahymena nuclear extracts. My 1979 notebooks record that, together with my technician San-San Chiou in the Department of Molecular Biology at UC Berkeley, over and over I made attempts to purify the telomeric proteins from nucleoli. Nucleoli are the tiny bodies within the Tetrahymena nucleus that harbor the actively transcribed rDNA minichromosomes. Fractionations after fractionations, mostly using sucrose gradients, were patiently performed by San-San. Then we scaled up the preparations – I purchased a huge industrial-sized Waring blendor that loomed like a leviathan on the laboratory bench. Tetrahymena cells were blended in order to disrupt them just enough to shake their nucleoli free from the rest of the nuclear contents. At one time my note-book laconically reported: “Respun only one-third of total … Waring blender broke.”
All these early efforts were to no avail. In retrospect, the experimental approach had been reasonable − to purify nucleoli, as being the most enriched form of telomeric chromatin known, then to digest them with micrococcal nuclease into fragments, the end ones containing the telomeric DNA terminal tracts and their bound proteins. Then, I would further fractionate these away from the rest of the chromatin by selective precipitation in potassium chloride solutions, or fractionate them by size on sucrose gradients. The goal was to see what protein(s) would co-purify, through these multiple fractionation steps, with the telomeric repeat tract DNA, which I followed through the multiple steps by its hybridization signal. But we were only able to obtain limited amounts of chromatin and binding factors, and we tried without success to get enough to identify any factors that might be specific to the rDNA ends. Looking back, I see that we were fighting against the numbers game – our detection methods were too frail, our preparation scale-ups too modest. Therefore, it was yeast genetics and approaches done by others that turned out to provide the next great leaps forward in understanding telomeric proteins. That I failed in this by my early attempts using Tetrahymena made me all the more determined, if anything, to use other approaches to try to understand the nature and biological significance of those strange-seeming repeated sequences at the ends of chromosomes.
I also recall that our failure to find telomeric proteins taught a lesson that became useful when it came to our work on Tetrahymena telomerase. As Carol Greider’s Nobel lecture describes, at one point the value of scaling up the telomerase activity preparations became evident to her. Thus, when Carol proposed the purchase of a very large glass column for preparative gel filtration chromatography, I was very willing to make this expensive-seeming purchase, ruefully recalling the past history of my too-pusillanimous scale-ups of Tetrahymena chromatin preparations.
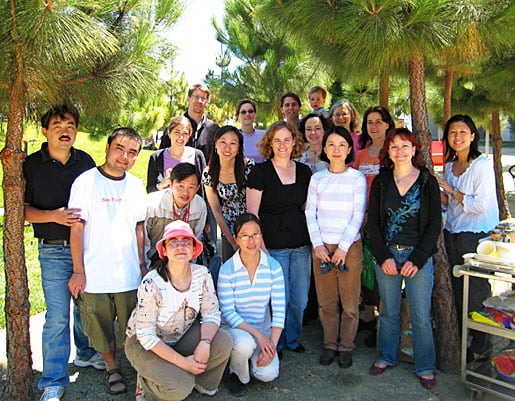
Figure 6. Mission Bay Laboratory Group BBQ, August 2007.
To the University of California San Francisco
I became a Full Professor at UC Berkeley in 1986 (after 8 years on the faculty of UC Berkeley), and in the same year a mother (our son Benjamin David was born in December 1986). By around 1989, I decided that as the long drive to Berkeley each day from our home in San Francisco made it difficult to pursue both science and our family life optimally, it was time to begin investigating alternatives. I settled upon a professorship at UCSF, and the move of my laboratory to UCSF’s Department of Microbiology and Immunology was accomplished in mid-1990. I have remained on the faculty of UCSF ever since. There, I have had the great good fortune to be able to keep delving into the nature and mechanisms of telomeres and telomerase. Together with colleagues in and out of UCSF and with my many talented students and postdoctoral fellows and technicians in my laboratory (Figure 6), I have been able to address the wondrous biological systems comprised of telomeres and telomerase. A fanciful depiction evoking both telomere dynamics and telomere researchers is shown in Figure 7. This painting, done by the artist Julie Newdoll in 2008, elicits the idea of a telomere as an ancient Sumarian temple-like hive, tended by a swarm of ancient Sumarian Bee-goddesses against a background of clay tablets inscribed with DNA sequencing gel-like bands.
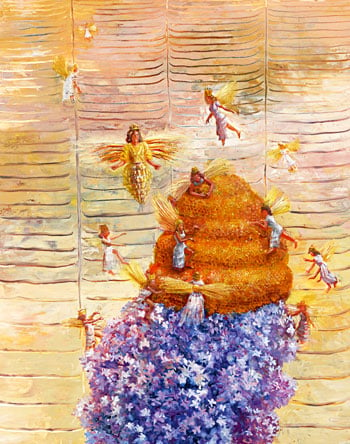
Figure 7. Julie Newdoll, Bee Goddesses.
Out of the Laboratory
In the 1990s my research’s implications for humans began to intrigue me, but with scientific research, faculty and Department Chair duties, family and many associated commitments, I had little time to indulge in delving into the philosophical and policy questions that can arise as science opens new possibilities. I served as President of the American Society for Cell Biology in 1998 and become more cognizant of the world of national science policy. Thus it was that in late 2001, the request to consider becoming a member of a newly created U.S. Federal Commission, the President’s Council on Bioethics, had a certain appeal. I felt that my knowledge of the relevant fields of science, and long experience in the world of research, would be useful contributions to the Council, a body that, as a Federal Commission, would be advisory on some matters of national science policy. A further appeal was the coincidence with my growing thinking about these issues. I reasoned that if I joined this Council, it would be an opportunity to contemplate some of these dimensions of research’s ramifications, and the possible reverberations of my own area of research.
Time for quiet contemplation of these and related questions in the abstract was not forthcoming. I understood from the beginning that the Bioethics Council would be occupied with publicly debated topics including human somatic cell nuclear transfer and embryonic stem cell research, as well as other topics less clearly defined at the outset of the council’s deliberations. I thought I should agree to serve on this Council because, as a seasoned scientist (particularly in cell and molecular biology), I might be able to offer perspectives that would be helpful in advising national scientific policy. I knew the topics upon which this Council, appointed by the George W. Bush administration, would advise would be politically charged ones. For this reason especially, I felt that a strong base of scientific fact and evidence would be particularly important, and useful advice in this vein was something that I could in fact offer to this advisory body.
I publicly made clear my views on some of the council’s recommendations, views that did not generally accord with those of the White House or with those of the Council’s Chair. After two years, I was informed by the Personnel Office of the George W. Bush White House that I would no longer be on this Council. This dismissal from the Council received quite a lot of public attention at the time. In the course of it, I was overwhelmed by the great many letters and communications I received. Almost without exception positive and supportive, they came from all over the United States and even from as far afield as a musician in London. His somewhat (to me) unexpected concern for science policy brought home to me how widespread is the wish among the public that science policy be informed by good scientific evidence. This entire episode was a broadening education. It reinforced my love of the searches for truth to which so many in research and academia aspire.
People Who Have Had Important Influences on My Life as a Scientist
I am indebted to so many individuals that I can only describe a few of them here. Growing up, three of my schoolteachers in particular encouraged my interests in biology and chemistry and mathematics, not least by letting me know that they believed in my abilities to succeed in these areas – Nan Hughes, Jenny Phipps and Len Stuttard.
As I embarked on research in biological science, my teachers, advisors and mentors − notably Frank Hird in Australia, Fred Sanger in Cambridge, England, and Joe Gall in the U.S.A. − not only imparted their scientific knowledge, visions and wisdom, but also their examples of how to be a scientist. In particular, a photograph of Joe Gall from 1999, although taken several years after I had been in his lab, captures in a succinct visual way some of Joe’s characteristics that influenced me when I was a member of his lab group (Figure 8). I took the photograph during a conference he was attending in Prague in the summer of 1999. During the conference a partial eclipse of the sun took place, and all the conference participants rushed out of the lecture hall to witness its progress. Joe is seen in the photograph demonstrating that it could be seen very simply and safely: All one had to do was hold a flat sheet of paper under a leafy bush so that the light, diffracted through the leaves onto the paper, caused to appear on the sheet of paper images of the “bite” being taken out of the disc of the sun by the moon passing in front of it. I recall that most of the conference participants had never seen this applied optics demonstration before. This photo evokes at once Joe Gall’s desire and ability to teach − by his use of a very striking demonstration to teach something new to the conference participants − and, not least, one glimpse of his wide knowledge encompassing optics and natural science in general.
Figure 8. Joseph G. Gall. Prague, 1999.
Like so many who are fascinated by chromosome behavior, I owe much to Barbara McClintock for her scientific findings. But in addition, Barbara McClintock also gave me a memorable lesson: in a conversation I had with her in 1977, during which I had told her about my unexpected findings with the rDNA end sequences, she urged me to trust my intuition about my scientific research results. This advice was surprising to me then, because intuitive thinking was not something that at the time I allowed myself to admit might be a valid aspect of being a biology researcher. I think her advice recognizes an important and sometimes overlooked aspect of the intellectual processes that underlie scientific research, and for me it had a liberating aspect to it. For this, also, I am very grateful to Barbara McClintock.
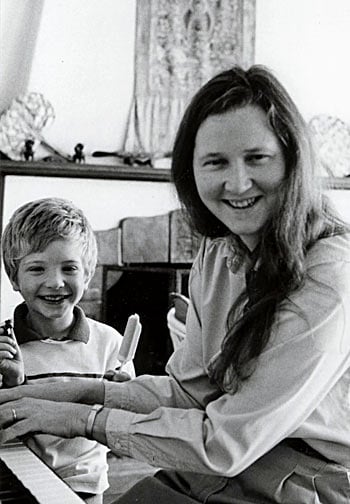
Figure 9. Elizabeth and her son, Ben, at the piano. Circa 1990.
My husband, John Sedat, himself an accomplished scientist, has always urged me to dig deeper into myself and find the reserves of strength I might not have tapped – his encouragement in this way has helped me through years of doing science. Our son Ben (Figure 9) inspired me to try to find ways of combining family and science, something that I have tried to convey to young scientists making their careers. Finally, my parents were both family physicians. From them I imbibed a sense of the importance of serving people kindly and as well as one can. I continue to believe that bioethics, done well and underpinned by the best available scientific evidence, can be an important part of our consideration, as a society, of the impact on people of scientific research in the biological sciences and medicine.
This autobiography/biography was written at the time of the award and later published in the book series Les Prix Nobel/ Nobel Lectures/The Nobel Prizes. The information is sometimes updated with an addendum submitted by the Laureate.
Nobel Prizes and laureates
Six prizes were awarded for achievements that have conferred the greatest benefit to humankind. The 12 laureates' work and discoveries range from proteins' structures and machine learning to fighting for a world free of nuclear weapons.
See them all presented here.