Advanced information
Scientific background:
Discoveries of receptors for temperature and touch (pdf)
Scientific background
Discoveries of receptors for temperature and touch
The 2021 Nobel Prize in Physiology or Medicine is awarded to David Julius and Ardem Patapoutian for their discoveries of thermal and mechanical transducers. The question of how we sense the physical world through somatic sensation has fascinated humankind for millennia. During the first half of the 20th century, it became clear that temperature and pressure activate different types of nerves in the skin. However, the identity of the molecular transducers responsible for detecting and converting heat, cold and touch into nerve impulses in the sensory nervous system remained a mystery until the discoveries awarded with this year’s Nobel Prize. David Julius wished to identify the cellular target of capsaicin, the pungent ingredient of chili peppers, as he believed this could provide fundamental insights into mechanisms of pain. He used a cDNA library from sensory neurons in a functional screen to look for a gene that could confer capsaicin sensitivity to cells that were normally unresponsive. The screen identified a cDNA encoding a novel ion channel (now called TRPV1) belonging to the family of transient receptor potential ion channels. Importantly, TRPV1 was shown to be activated by temperatures perceived as painful. Following the discovery of TRPV1, David Julius and Ardem Patapoutian independently made another important advance with the discovery of TRPM8, a related cold-sensitive receptor. Several additional TRP-receptors were subsequently identified and shown to transduce thermal information in the somatosensory system. Thus, the seminal discovery of TRPV1 by David Julius opened the door to a molecular understanding of thermosensation. Ardem Patapoutian used a functional screen of candidate genes expressed in a mechanosensitive cell line to identify ion channels activated by mechanical stimuli. Two mechanically-activated ion channels, named PIEZO1 and PIEZO2, were identified and shown to represent an entirely novel class of ion channels functioning as mechanical sensors. Importantly, Patapoutian also demonstrated that PIEZO2 is the major mechanical transducer in somatic nerves and is required for our perception of touch and proprioception. In further work, he uncovered central roles of PIEZO1 and PIEZO2 for many additional physiological functions. The work by the two laureates has unlocked one of the secrets of nature by explaining the molecular basis for sensing heat, cold and mechanical force, which is fundamental for our ability to feel, interpret and interact with our internal and external environment.
From moving particles to nerve conduction
Somatic sensation has fascinated humankind for millennia. In an attempt to explain how we react to heat, the 17th century philosopher René Descartes depicted that particles of fire pulled a thread between the skin and brain [1]. In the 1880s, distinct sensory spots on the skin were shown to react to specific stimuli, such as touch, heat or cold, indicating that different stimuli activate different types of nerves [2, 3]. Three previous Nobel Prizes in Physiology or Medicine have significantly advanced our understanding of the somatic sensory nervous system. In 1906, Camillo Golgi and Santiago Ramón y Cajal received the Nobel Prize for their work on the structure of the nervous system, which included an anatomical description of the somatosensory system. Sir Charles Sherrington and Edgar Adrian received the Nobel Prize in 1932 for their discoveries regarding the function of neurons, including a description of somatosensory neurons. In 1944, Joseph Erlanger and Herbert Spencer Gasser received the Nobel Prize for their discoveries related to the differentiated functions of single somatosensory nerve fibers. These discoveries established important principles for the propagation of action potentials along skin and muscle sensory nerve fibers. The discovery of different nerve fiber types with distinct conduction velocities, activation thresholds and refractory periods, made it possible to link specific nerve fiber types to different somatosensory modalities, such as proprioception (the sense of our body’s movement and position in space), touch and temperature sensation. However, fundamental questions remained unsolved: What is the nature and molecular identity of the receptors that can sense temperature and touch and how can those sensors convert stimuli into action potentials within the somatosensory nerve fibers?
Sensing the environment
The ability to sense and adapt to the environment is essential for survival in all organisms. For example, bacteria adapt to changes in osmotic force through the activation of mechanosensitive ion channels enabling them to survive when trapped in rainwater [4, 5]. In humans and other animals, somatic sensation arises from the body surface or internal organs and endow us with the sense of touch, proprioception, pain and temperature. These are vital functions allowing organisms to continuously adapt to changes in the external and internal environment.
Somatic senses involve peripheral sensory pathways that detect and convert objective information about the physical properties of various stimuli (e.g. mechanical and thermal) into electrical signals that are conveyed to the central nervous system. The sense of touch, initiated by the detection of mechanical force, provides us with the recognition of texture, size and shape of objects as well as tactile and vibration sensitivity. This sense, for example, allows us to recognize the softness of a pillow, gentle caress of the skin or the feeling of a breeze. The capacity of discrimination of perceptual qualities arises from unique functions of a variety of sensory neurons involved in touch sensation. Thus, different stimuli, such as skin indentation, skin stretch, hair deflection or vibration, activate different types of sensory neurons [6]. The somatosensory system also conveys information on limb movement and position in space (i.e., proprioception), allowing us to sense when an arm or a leg is stretched or folded.
Another aspect of somatic sensation relates to pain induced by noxious stimuli that activate a class of polymodal nerve fibers (called nociceptors) in response to strong mechanical force and painful heat [7, 8]. These nociceptors transmit information on potentially harmful changes in our physical environment, e.g. when touching a hot stove or holding a hand in ice water. Accordingly, pain represents an essential protective mechanism that prevents tissue damage through reflex reactions.
Apart from providing conscious awareness about our body and its surroundings, the somatosensory system is also essential for tasks that we perform effortlessly and without much thought. For example, when drinking a glass of water, sensory neurons convey information about the weight, size, texture and temperature of the glass so that an appropriate grip strength can be applied and movements coordinated when taking a sip. Similarly, the seemingly simple task of walking also requires a continuous flow of sensory information to coordinate and correct limb move-ments and maintain balance.
Through their ground-breaking work the 2021 Nobel Prize laureates have identified the long sought molecular transducers for sensing temperature and mechanical force. Their discoveries have unlocked one of the remaining mysteries of how somatic sensation enables us to feel and interact with the physical world.
The discovery of thermosensitive ion channels for thermal sensation
A prelude
Capsaicin (8-methyl-N-vanillyl-6-nonenamide), the active component of chili peppers, gives the burning sensation when eating spicy food. Studies on the chemical provided important insights that opened for the discovery of the first heat-sensitive receptor. Studies in the 1950s showed that sweating of the head is induced when hot peppers are in contact with the mouth or lips, a phenomenon called gustatory sweating [9]. During the following decades, capsaicin was found to act on sensory nerves [10] and to induce ionic currents [11-14]. In parallel, it was also shown that noxious heat produced activation of ion channels in sensory neurons [15, 16]. However, it was not fully clear if the channel itself was the transducer of thermal energy.
The discovery of TRPV1 as a thermosensitive ion channel in sensory neurons
In the late 1990s, David Julius at the University of California, San Francisco, pursued a project to identify the receptor for capsaicin. He thought that understanding the action of capsaicin could provide insights into pain signaling. Together with a postdoctoral fellow, Michael J. Caterina, Julius decided to conduct an unbiased functional screen based on the assumption that a single gene can confer capsaicin sensitivity in cells that are normally insensitive to capsaicin. To find this putative gene, Julius and coworkers made a cDNA library from rodent dorsal root ganglia that contain the cell bodies of the capsaicin-activated sensory neurons. Capsaicin-insensitive cells were transfected with batches of these cDNAs and eventually a single cDNA clone was isolated that could confer responsiveness to capsaicin [17] (Figure 1A). The isolated gene was predicted to encode an integral membrane protein with six transmembrane domains and a homology search revealed that it belonged to the superfamily of transient receptor potential (TRP) cation channels [18, 19]. Julius continued to functionally characterize the TRPV1 receptor (at the time called vanilloid receptor 1, VR1) by ectopic expression in cells and found that the capsaicinevoked electrophysiological properties resembled those of channels found in native sensory neurons. He also noted that the transfected cells became sensitive to cytotoxic effects induced by capsaicin and that the capsaicin-evoked responses could be blocked with an antagonist. Further characterization showed that TRPV1 was expressed in nociceptive dorsal root ganglion neurons, thus providing an explanation for the selective actions of capsaicin on these cells (Figure 1B). While exploring the physiology of TRPV1, Julius examined its sensitivity to elevated temperature and found a pronounced activation by heat leading to cellular Ca2+ influx. Direct measurement of currents using patch-clamp recordings revealed a specific heat-evoked membrane current with properties similar to those of sensory neurons. Furthermore, TRPV1 had an activation threshold (above 40°C) close to the psychophysical threshold for thermal pain (Figure 1C).
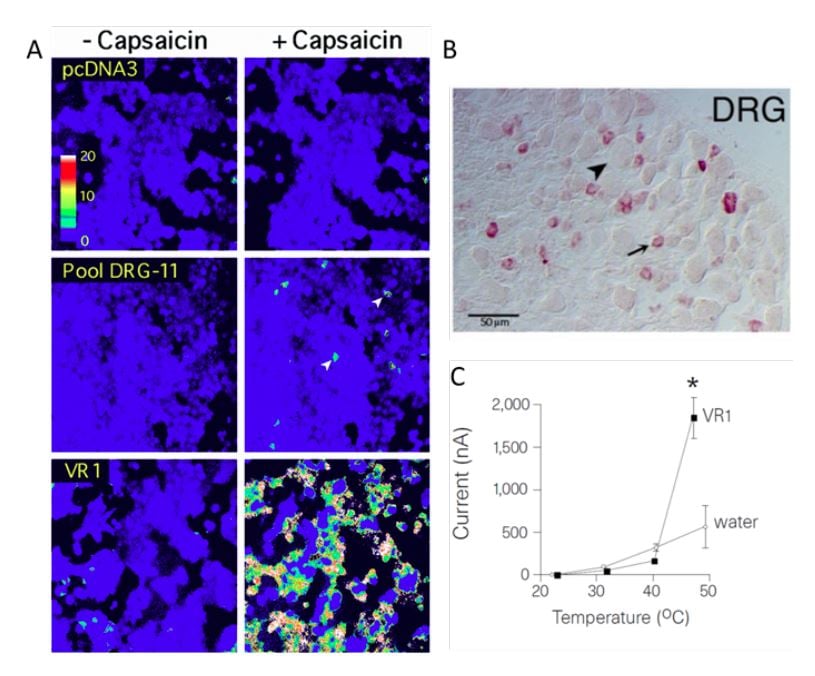
Figure 1. From Caterina et al., 1997 [17]. A) HEK293 cells transfected with pools of cDNA clones. In pool DRG-11 some cells responded to capsaicin. After iterative assaying, a clone corresponding to TPRV1 (VR1) was identified. B) Expression of TRPV1 in dorsal root ganglion neurons. C) The temperature sensitivity of TRPV1 expressed in oocytes is in the noxious range, above 40°C.
Shortly after identifying TRPV1, Julius went on to show that heat directly activates this channel in the absence of other factors, and that it acts as a molecular integrator of painful heat stimuli and chemical stimuli [20]. TRPV1 was expressed in unmyelinated nociceptive neurons, but not in neurons involved in proprioception, touch and pressure sensation, consistent with its role as a transducer of noxious heat. The role of TRPV1 as the only receptor activated by capsaicin and its essential role for transducing the nociceptive, inflammatory and hyperthermic effects of capsaicin was subsequently established in mice. TRPV1 is also required for inflammatory heat hyperalgesia in mice [21, 22]. Recent clinical studies of selective TRPV1 antagonists confirms a major role for this ion channel for sensing noxious heat in humans [23, 24]. The seminal discovery of TRPV1 as the capsaicin- and heat-activated ion channel in 1997 opened the field and represented a landmark achievement in our quest to understand the molecular and neural basis for thermal sensing.
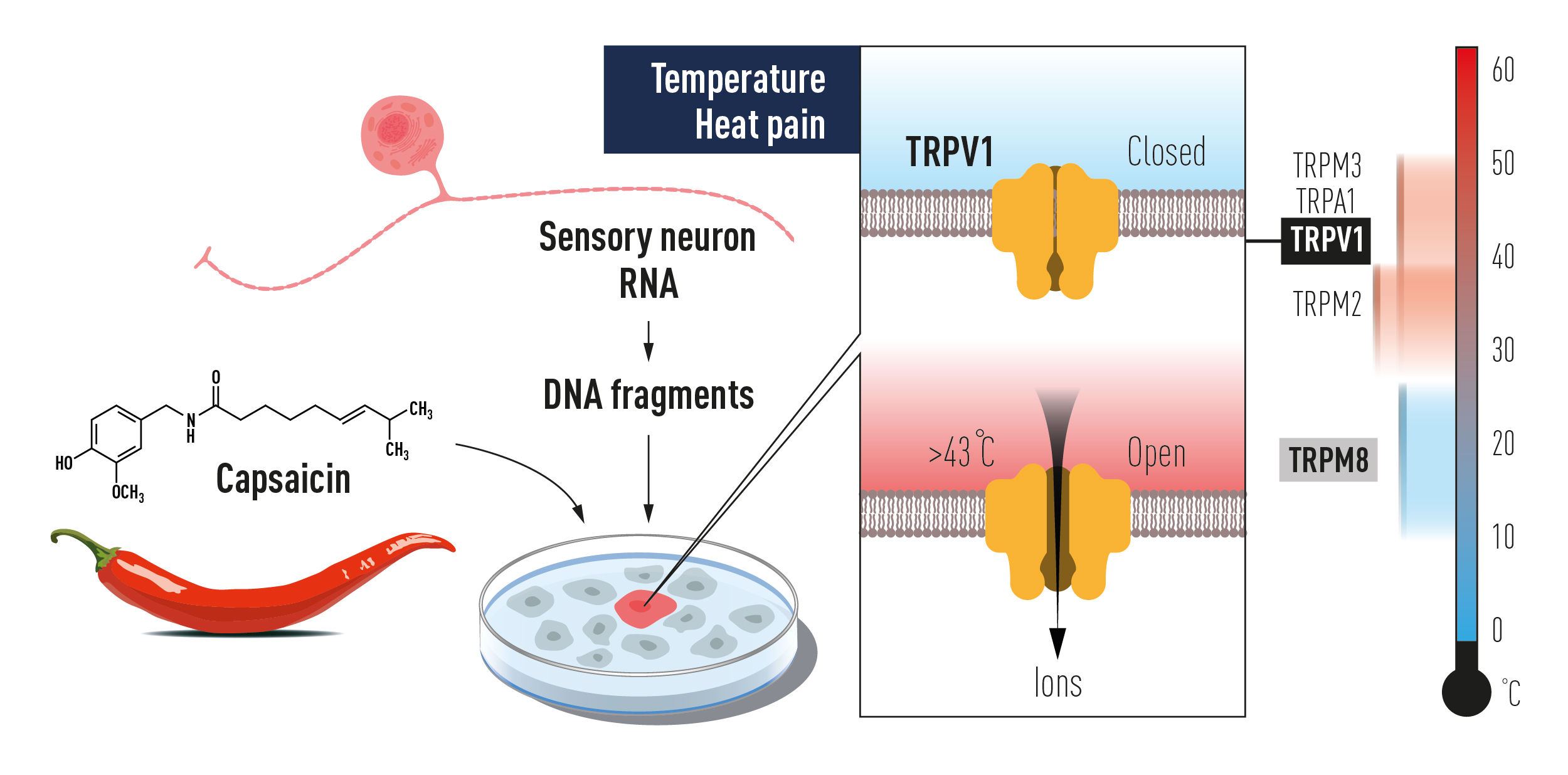
Figure 2. The discovery of TRPV1 using a gain of function screen of genes expressed in sensory neurons for reconstitution of capsaicin responsiveness in a non-responsive cell line. This paved the way to the unravelling of additional temperature-sensing TRP receptors, which together code for temperature sensation.
The overall transmembrane topology and subunit architecture of TRPV1 and other TRP channels is similar to voltage-gated sodium and potassium channels [25, 26]. The structure of TRPV1 has been determined by cryo-electron microscopy in a collaboration between the Julius and Yifan Cheng laboratories. TRPV1 seems to have two gates that form two prominent physical constrictions at either end of the cation-conducting pore [27, 28]. Capsaicin binds a pocket in TRPV1 located deep in the membrane close to the cytoplasmic side. A recent structural study showed that noxious heat produces two conformational gating transitions in capsaicin-bound TRPV1 channels [29]. The first transition primes the channel for opening, while the second leads to channel opening. The structural studies of TRPV1 channels have provided important insights into mechanisms for their ion permeation, ligand recognition and gating, but the mechanisms for their activation by heat are not fully understood at the structural level. sensation.
The sensation of noxious heat
Whereas TRPV1 was found to have a critical role for the increased sensitivity to heat during inflammation, it was evident that other heat sensitive receptors must exist because animals lacking Trpv1 showed only a minor loss of acute noxious heat sensation [21, 22, 30, 31]. In 2011, Voets’ group identified TRPM3 as a second sensor for noxious heat in Trpv1 knockout mice [32]. However, the inactivation of both Trpv1 and Trpm3 in mice blunted, but did not eliminate, reflex responses to noxious heat. The attention therefore turned to a third TRP channel, TRPA1, which had been discovered in 2004 as a transducer for pungent chemicals independently by Julius and Patapoutian laboratories [33, 34]. TRPA1 is involved in the detection of a wide variety of noxious external stimuli, such as active compounds in mustard oil, horseradish, cinnamon, garlic, cloves, and ginger, as well as lipid compounds, environmental irritants and other chemicals [33-38]. The TRPA1 ion channel is polymodal and can be activated by various chemical substances, as well as by cold and heat in a way that differ between mammalian species [39]. Because of this complexity, the role for TRPA1 as a thermosensor in mammalian sensory neurons was debated [36, 40-43]. The question of which ion channels contribute to noxious heat sensation in mice was resolved when Voets` group showed that it depends on a triad of ion channels, namely TRPV1, TRPM3 and TRPA1 [44].
The sensation of cold
Non-noxious cold sensation in humans and mice starts around 28°C and has a remarkable precision detecting changes as small as 0.5°C in skin temperature [43, 45, 46]. In 2002, the sensory transducer for cold was independently discovered by the Julius and Patapoutian laboratories [47, 48] in functional screens based on the assumption that menthol, a natural compound that elicits the sensation of innocuous coolness in humans, binds an ion channel that is activated by low temperature. Both groups identified TRPM8, yet another member of the TRP superfamily, and found that it is activated by low temperature in a heterologous expression system at a temperature range at which humans perceive innocuous cold [47, 48]. Consistent with these findings, Julius, Patapoutian and other groups independently found that deletion of Trpm8 in mice causes clear deficits in sensation of innocuous cold [49-51]. The discovery of TRPM8 as a cold sensor placed the TRP superfamily at the center stage of thermal somatosensation and paved the way to the identification of additional TRP channels responsible for thermal sensation.
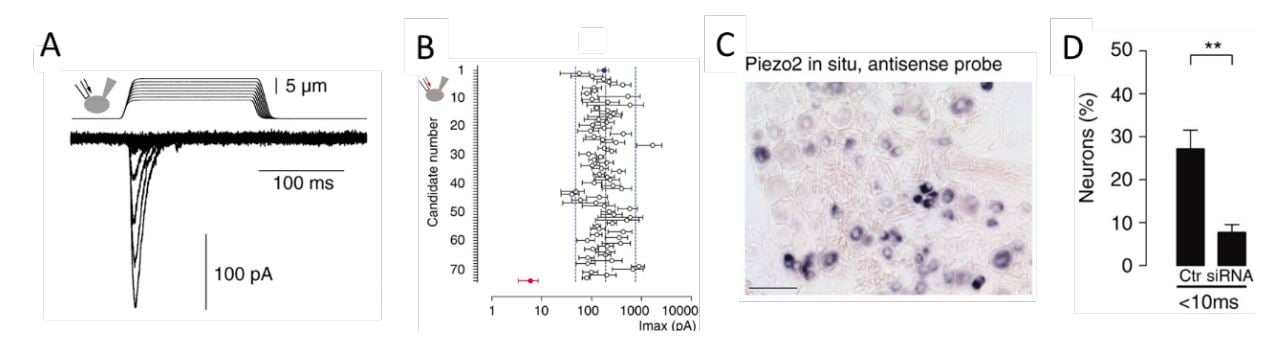
Figure 3. From Coste et al., 2010 [64]. A) Mechanosensitive currents in Neuro2A cells. B) Average amplitude of currents in Neuro2A cells with knockdown of candidate genes. C) Expression of PIEZO2 in a subset of dorsal root ganglion sensory neurons. D) Loss of responsiveness to mechanical stimulation following knockdown of PIEZO2 expression in dorsal root ganglion neurons.
The sensation of warmth
The detection of changes in skin temperature is very precise, with changes of warmth detected with perceptual thresholds of around 1°C in humans [45, 52]. Similar to humans, mice also detect subtle changes of temperature in the warm range and this remarkable sensitivity to changes in temperature relies on TRP channels. While TRPV1 was initially considered only as a receptor for noxious heat, subsequent studies unexpectedly found that TRPV1 also contributes to detection of innocuous warmth [43]. Furthermore, Peter McNaughton’s group identified another TRP channel, TRPM2, as a potential warmth sensor. Deletion of the gene for this channel in mice resulted in deficits in the sensation of innocuous warm temperatures in the range (33-38°C) [53]. Recently, it was found that discrimination between warm and cool temperatures depends on the simultaneous activation of warmth-sensing and inhibition of cold-sensing nerve fibers [54]. The emerging picture is that TRPV1, TRPA1, TRPM2 and TRPM3 ion channels collectively act as warm sensors, but that the warmth sensation is reliably signaled only when the activity in TRPM8 containing cold-sensing nerve fibers is simultaneously suppressed by warm temperatures [54].
In conclusion, the seminal discovery of TRPV1 initiated intense investigation that has now firmly established the critical role of TRP channels for thermal sensation (Figure 2). The findings show that several TRP channels gated at different temperature ranges act together to code for temperature and heat-induced pain in the somatosensory nervous system. At present, important roles for TRPV1, TRPA1, TRPM3, TRPM2 and TRPM8 in temperature sensing have been experimentally established. Future studies will likely provide additional insights in this active research field.
The discovery of a vertebrate mechanosensitive ion channel
A prelude
The existence of a vertebrate mechanosensitive ion channel was suggested more than 40 years ago based on data showing rapid membrane depolarizations following mechanical stimulation of cochlear hair cells in frogs [55]. However, the existence of mechanosensitive channels was not firmly established until the late 1980’s when Ching Kung and Boris Martinac identified and characterized such channels in Escherichia coli [5, 56, 57]. The identified channels act as force sensors for adaptation to environmental changes and have an essential role as even a mild change in osmolarity cause bacteria to lyse in the absence of mechanosensitive channels [58].
The finding of a mechanosensitive ion current in rat dorsal root ganglion neurons suggested that touch sensation in vertebrates also rely on activation of a mechanosensitive ion channel [59]. However, orthologs of candidate channels previously identified in Caenorhabditis elegans and Drosophila melanogaster did not seem to play key roles for touch sensation in vertebrates [60]. Furthermore, several potentially mechanosensitive channels identified in vertebrates were not confirmed as critical touch receptors in functional experiments [60-63]. The identity of the receptors for somatic mechanosensation in mammals thus remained an enigma.
The discovery of PIEZO2 as a mechanosensitive ion channel for touch and proprioception
Ardem Patapoutian at Scripps Research, California developed a novel screening approach to search for the elusive receptor for mechanosensation in mammals. Together with the postdoctoral fellow Bertrand Coste, he identified an intrinsically mechanosensitive cell line, called Neuro2A, by using brief and rapid indentation of the plasma membrane in combination with patchclamp recording to detect any possible current induced by the mechanical force (Figure 3A) [64]. Once the mechanosensitive Neuro2A cell line was identified, Patapoutian performed global expression analysis and identified 72 candidate genes predicted to encode proteins with at least two membrane-spanning domains, which included known ion channels and proteins of unknown function. The candidate genes were silenced oneby-one by RNA interference and the transfected cells were tested to determine whether the application of mechanical force resulted in a current that could be recorded using patch-clamp. Knockdown of the final gene on the list, previously known as FAM38A, eliminated the mechanicallyactivated current and the corresponding protein was named PIEZO1 from the Greek word “piesi” meaning pressure (Figure 3B, red data point). Patapoutian proceeded to show that ectopic expression of PIEZO1 made human embryonic kidney cells (HEK-293) mechanosensitive, as pressure applied to the plasma membrane induced a large current in these cells. A second mechanosensitive channel, named PIEZO2, was subsequently discovered by sequence homology. The newly identified PIEZO channels belonged to a previously unknown protein family present in vertebrates and many other eukaryotes. PIEZO2, but not PIEZO1, was found to be expressed in dorsal root ganglion sensory neurons (Figure 3C) and knockdown of PIEZO2 abolished the mechanosensitivity of these sensory neurons (Figure 3D).
Direct evidence that PIEZO2 is the sensor for light touch was established in 2014, when Patapoutian and other researchers demonstrated that Merkel cells display a PIEZO2-dependent current evoked by fast touch- and that this current is sufficient to sustain action potential firing in tactile sensory afferents [65-67]. However, consistent with a “tworeceptor-site hypothesis” [68], stating that both Merkel cells and innervating sensory neurons are mechanosensitive, major components of touch sensation remained in the absence of Merkel cell activity. In a later study in 2014, Patapoutian engineered mice that lack PIEZO2 in both Merkel cells and adult sensory neurons. These mice were profoundly deficient in light touch sensation without impairment of thermosensation [69]. Consistent with these findings, humans with lossof-function mutations in PIEZO2 also display profound deficits in touch sensation, including texture discrimination, hair deflection as well as tactile and vibration sensitivity [70-73].
The Patapoutian group also demonstrated that PIEZO2 is the principal transduction channel for proprioception in mice as its absence results in severely uncoordinated body movements and abnormal limb positions [74]. Similar observations were also made in humans lacking functional PIEZO2 [70, 72]. The groundbreaking discovery of PIEZO proteins as excitatory ion channels directly gated by mechanical force has revolutionized the field of neuroscience by providing a molecular basis for mechanosensation.
PIEZO proteins represent an entirely new class of vertebrate mechanosensitive channels without any resemblance to previously known ion channel families. They are the largest transmembrane ion channel subunits identified to date, composed of 2,500 amino acids and display a unique 38transmembrane helix topology. Work from Patapoutian and other laboratories has revealed the high resolution structure of PIEZO1 and PIEZO2 and has shown that these channels form homotrimeric structures with a central ionconducting pore and three peripheral large mechanosensing propeller-shaped blades [7578]. The three blades curve out and up creating a nano-bowl configuration in the surface of the cell membrane [77-79]. When a mechanical force is applied to the membrane, the curved blades flatten out and lead to the opening of the central pore. The propeller-like structure with curved blades generate a large in-plane membrane area expansion, which likely explain the exquisite mechanosensitivity of PIEZO channels [77, 80]. However, the exact mechanisms whereby mechanical force opens the central pore are still not completely understood. Through their mechanosensitivity, PIEZO channels serve as versatile mechanotransducers in many cell types and convert mechanical force into electrochemical signals (Figure 4).
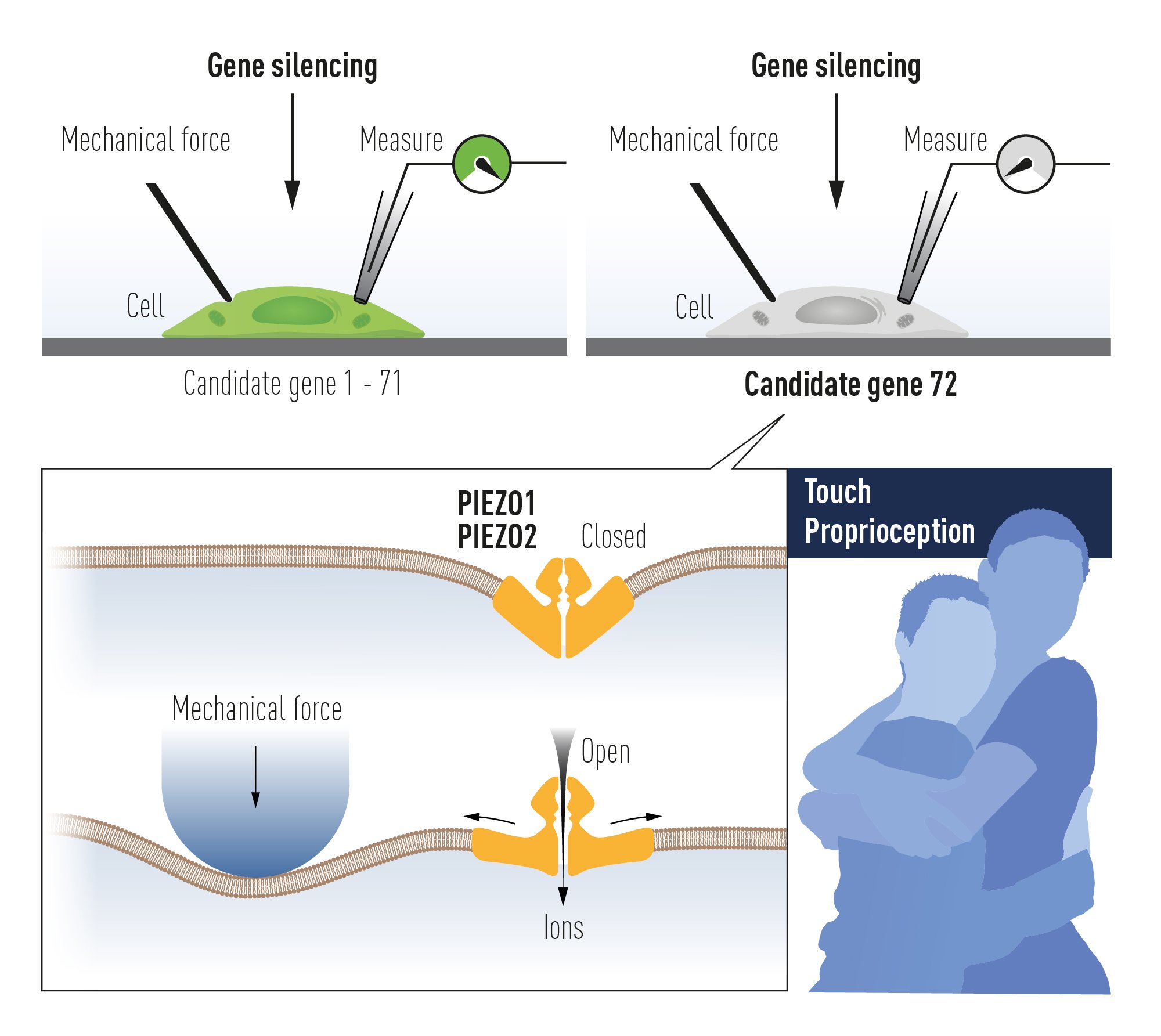
Figure 4. The discovery of PIEZO channels using gene silencing of 72 candidate genes in a mechanosensitive cell line and searching for a loss of mechanoreception. This paved the way to the unravelling of PIEZO2 as the mechanoreceptor for touch and proprioception, senses used for example in a hug.
PIEZOs as mechanosensors in internal organs
In 1938, the Nobel Prize in Physiology or Medicine was awarded to Corneille Heymans for discovering the sensory function of the vagus nerve in reflexes, including the respiratory reflex as described by Hering and Breuer. In a collaboration with other research groups, the Patapoutian lab showed that deletion of Piezo2 in visceral nodose (vagal) sensory ganglion neurons of adult mice led to impairment of the HeringBreuer reflex and increased the respiratory tidal volume. Furthermore, deletion of Piezo2 during development caused respiratory distress and death at birth [81]. Thus, these studies show that PIEZO2 channels present on pulmonary stretch receptors in the wall of bronchi and bronchioles are activated by large inspirations and initiate a reflex protecting the lung from over-inflation.
Studies of the glossopharyngeal and vagus nerves by Corneille Heymans also identified the baroreflex. Patapoutian and collaborators demonstrated that the arterial baroreflex, which continuously monitors and maintains blood pressure, relies on both PIEZO1 and PIEZO2 present in nodose (vagus) and petrosal (glossopharyngeal) sensory neuron ganglia. Mice lacking Piezo1 and Piezo2 display a labile hypertension and have increased blood pressure variability, similar to the finding in humans with baroreflex failure [82, 83].
PIEZO2 is also important in the gastrointestinal tract where the enterochromaffin cells are inherently mechanosensitive and release hormones and paracrine signaling molecules in response to mechanical stimulation by gastrointestinal luminal content [84, 85]. Furthermore, PIEZO2 is the mechanosensor in urothelial cells and bladder sensory neurons. Mice and humans lacking functional PIEZO2 therefore have impaired urinary bladder control [86].
PIEZO1 plays an important role as a sensor of mechanical forces in endothelial cells, red blood cells, and osteoblasts in mice. The sensing of shear-stress in endothelial cells is important for the formation of blood vessels during development, for angiogenesis in adult tissues as well as for the regulation of vascular tone [87-91]. In addition to its role in blood vessel integrity, PIEZO1 is also present in red blood cells where it is involved in cell volume homeostasis. Piezo1 deletion in mice leads to overhydration of red blood cells whereas a chemical compound called Yoda1 that activates PIEZO1 leads to dehydration of red blood cells [92]. Strain induced by mechanical forces is linked to skeletal remodeling, and in mice the mechanical load-dependent bone formation relies on PIEZO1 in osteoblasts, where it functions as a mechanotransducer [93-95].
Relevance for humans and medicine
Behavioral studies of animal models have been critical for our understanding of the molecular mechanisms underlying temperature and touch. However, it is impossible to fully recapitulate human somatic sensations in animals and we cannot truly know whether a rodent is sensing touch or proprioception by merely studying its reactions. Studies in human subjects with genetic mutations in TRP and PIEZO channels have therefore provided significant insights into the roles of these channels in transducing temperature, pain, touch, vibration and proprioception.
Human genetics and temperature sensing TRP channels
There are several genetic “TRP channelopathies” in humans. Among the temperature sensing TRP channels, an autosomal dominant TRPA1 channelopathy named Familial Episodic Pain Syndrome type 1 is caused by a point mutation in TRPA1 and manifested by episodes of debilitating upper body pain triggered by cold, fasting and physical stress [96]. Several studies have investigated the role of single nucleotide polymorphisms (SNPs) in TRP channel genes and identified an association of TRPA1 710G>A with neuropathic pain and a paradoxical heat sensation. In addition, TRPV1 1911A>G has been associated with cold hypoalgesia [97], while several other SNPs in TRPV1 alters the sensitivity to capsaicin.
Mutations in PIEZO2 profoundly impact the sense of touch, vibration and proprioception
Patapoutian and several other researchers have reported that mutations in the PIEZO2 gene underlie several genetic disorders manifested by altered sensations of touch, vibration and proprioception. Whereas mice lacking PIEZO2 die at birth due to respiratory distress, humans with biallelic loss-of-function mutations survive. Lossof-function mutations in the PIEZO2 gene result in an autosomal recessive condition named distal arthrogryposis (DA) with congenital contractions in multiple joints of fingers, feet and toes along with impaired proprioception and touch (DAIPT) [7072, 98]. As more families with DAIPT from different parts of the world have been reported and the phenotypic manifestations are better understood, the alternative name PIEZO2 deficiency syndrome has been coined. Patients with PIEZO2 deficiency syndrome exhibit greatly attenuated proprioception, sense of touch and vibration. This results in sensory ataxia, dysmetria, gait difficulties, muscle weakness and atrophy, scoliosis, hip dysplasia and progressive skeletal contractures. These patients also have deficiencies of interoceptive sensations from the lung leading to perinatal respiratory distress and the bladder causing impairments in urination [86]. These patients fail to develop sensitization and painful reactions to touch after skin inflammation, suggesting a critical role for PIEZO2 is tactile allodynia [99]. However, patients with PIEZO2 deficiency syndrome have intact sense of innocuous deep pressure [100] and noxious mechanical pain responses [70, 99, 101].
Gain-of-function mutations of PIEZO2 cause autosomal dominant DA type 5, a form with prominent oculomotor symptoms [102, 103]. Other autosomal dominant mutations cause DA type 3, also called Gordon syndrome [103, 104], which is distinguished from other distal arthrogryposes by the presence of short stature and cleft palate. PIEZO2 gain-of-function mutations have also been reported in Marden-Walker Syndrome, a DA form characterized by psychomotor retardation, micrognathia and kyphoscoliosis [103].
Mutations in PIEZO1 impair physiological functions of red blood cells and the development of the lymphatic system
Mice lacking PIEZO1 die embryonically, whereas humans with biallelic loss-of-function mutations survive. Patapoutian and several other researchers have shown that biallelic loss-offunction mutations or compound heterozygous mutations in PIEZO1 causes an autosomal recessive, unique form of generalized lymphatic dysplasia, known as lymphatic malformation 6 [105, 106]. It is characterized by general facial and limb lymphoedema and indicate that PIEZO1 is involved in the development of the corresponding lymphatic structures.
Gain-of-function mutations in PIEZO1 leads to an autosomal dominant hemolytic anemia named dehydrated hereditary stomatocytosis (DHS) or hereditary xerocytosis [107-109]. This anemia is characterized by macrocytosis, the presence of stomatocytes and dehydration of red blood cells. The dehydration is caused by a defect in cellular cation content and some patients have a pseudohyperkalemia. Point mutations underlying DHS have been found at highly conserved residues in the C-terminal half of the PIEZO1 protein.
Patapoutian has shown that a gain-of-function E756del PIEZO1 allele causes dehydration of red blood cells and decrease the risk for severe Plasmodium falciparum infection [110, 111]. This allele is highly prevalent and enriched in Africans, raising the possibility that it is under positive selection due to malaria. The E756del PIEZO1 allele is also linked to increased red blood cell turnover and elevated serum iron in African individuals [112].
Concluding remarks
The groundbreaking discoveries of the TRPV1, TRPM8 and PIEZO channels by this year’s Nobel Prize laureates have allowed us to understand how heat, cold and mechanical force are sensed and transformed into nervous impulses that enable us to perceive and adapt to the world around us. The TRP channels are central for our ability to perceive temperature. The PIEZO2 channel endows us with touch and proprioception. TRP and PIEZO channels also contribute to numerous additional physiological functions depending on sensing temperature or mechanical stimuli (Figure 5). Intensive ongoing research originating from this year’s Nobel Prize awarded discoveries are focused on elucidating the functions of these receptors in a variety of physiological processes and to develop treatments for a wide range of disease conditions, including chronic pain.
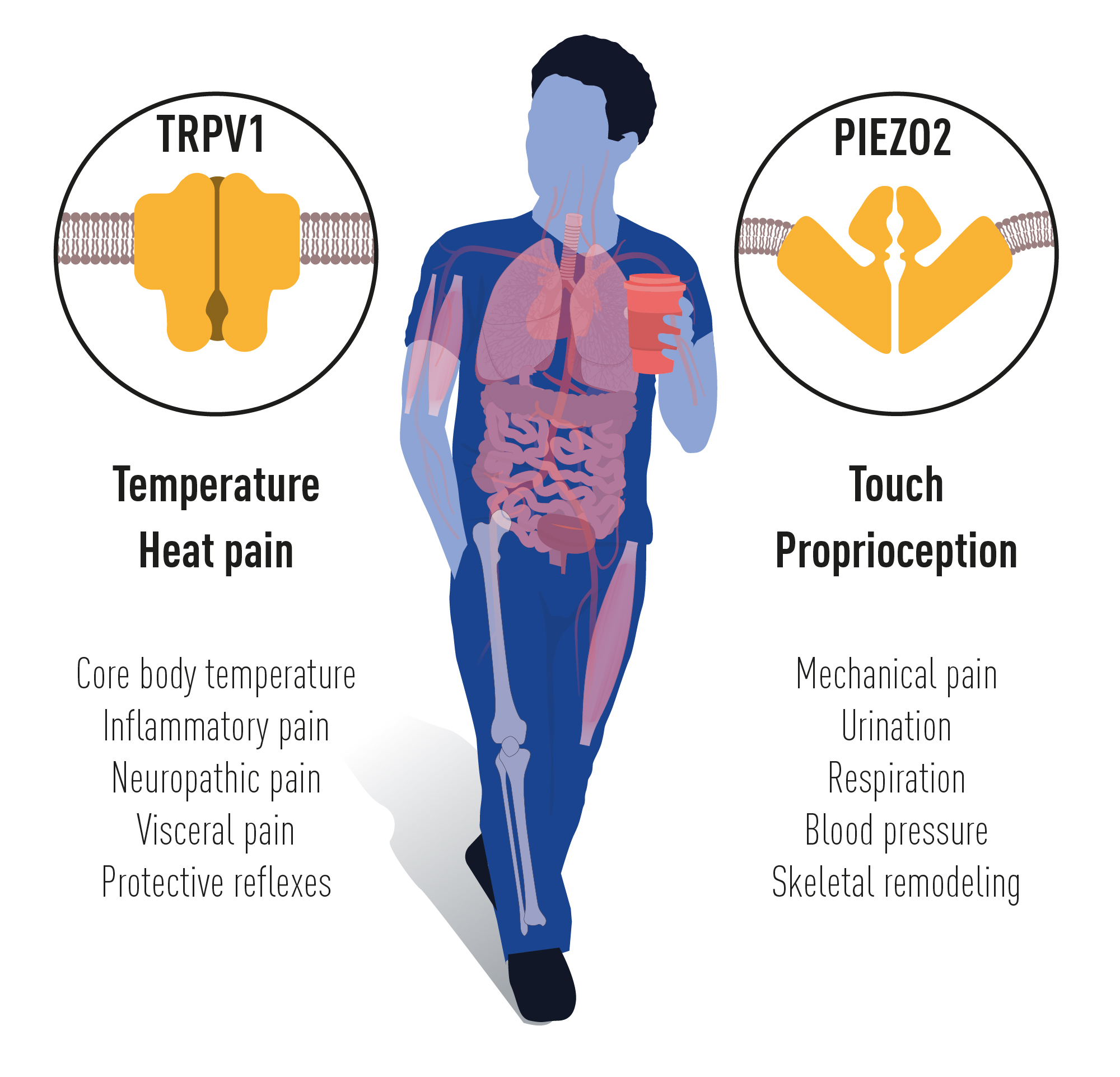
Figure 5. TRPV1 and PIEZO2 channels endow us with the sensation of temperature, heat pain, touch and
proprioception. Numerous additional physiological functions rely on the temperature and mechanically
sensitive TRP and PIEZO channels.
Patrik Ernfors, PhD, Professor at Karolinska Institutet
Member of the Nobel Committee
Abdel El Manira, PhD, Professor at Karolinska Institutet
Member of the Nobel Committee
Per Svenningsson, MD PhD, Professor at Karolinska Institutet
Member of the Nobel Committee
Illustrations: Mattias Karlén
The Nobel Assembly, consisting of 50 professors at Karolinska Institutet, awards the Nobel Prize in Physiology or Medicine. Its Nobel Committee evaluates the nominations. Since 1901 the Nobel Prize has been awarded to scientists who have made the most important discoveries for the benefit of humankind.
Nobel Prize® is the registered trademark of the Nobel Foundation
References
- DeLeo, J.A., Basic science of pain. J Bone Joint Surg Am, 2006. 88 Suppl 2: p. 58-62.
- Melzack, R. and P.D. Wall, On the nature of cutaneous sensory mechanisms. Brain, 1962. 85: p. 331-56.
- Norrsell, U., S. Finger, and C. Lajonchere, Cutaneous sensory spots and the “law of specific nerve energies”: history and development of ideas. Brain Res Bull, 1999. 48(5): p. 457-65.
- Kung, C., A possible unifying principle for mechanosensation. Nature, 2005. 436(7051): p. 647-54.
- Martinac, B., M. Buechner, A.H. Delcour, J. Adler, and C. Kung, Pressure-sensitive ion channel in Escherichia coli. Proc Natl Acad Sci U S A, 1987. 84(8): p. 2297-301.
- Handler, A. and D.D. Ginty, The mechanosensory neurons of touch and their mechanisms of activation. Nat Rev Neurosci, 2021. 22(9): p. 521-537.
- Bessou, P. and E.R. Perl, Response of cutaneous sensory units with unmyelinated fibers to noxious stimuli. J Neurophysiol, 1969. 32(6): p. 1025-43.
- Burgess, P.R. and E.R. Perl, Myelinated afferent fibres responding specifically to noxious stimulation of the skin. J Physiol, 1967. 190(3): p. 541-62.
- Lee, T.S., Physiological gustatory sweating in a warm climate. J Physiol, 1954. 124(3): p. 528-42.
- Jancsó, G., E. Kiraly, and A. Jancsó-Gábor, Pharmacologically induced selective degeneration of chemosensitive primary sensory neurones. Nature, 1977. 270(5639): p. 741-3.
- Dray, A., J. Bettaney, and P. Forster, Actions of capsaicin on peripheral nociceptors of the neonatal rat spinal cord-tail in vitro: dependence of extracellular ions and independence of second messengers. British Journal of Pharmacology, 1990. 101(3): p. 727-733.
- Marsh, S.J., C.E. Stansfeld, D.A. Brown, R. Davey, and D. McCarthy, The mechanism of action of capsaicin on sensory C-type neurons and their axons in vitro. Neuroscience, 1987. 23(1): p. 275-89.
- Szallasi, A. and P.M. Blumberg, Specific binding of resiniferatoxin, an ultrapotent capsaicin analog, by dorsal root ganglion membranes. Brain Res, 1990. 524(1): p. 106-11.
- Wood, J.N., J. Winter, I.F. James, H.P. Rang, J. Yeats, and S. Bevan, Capsaicin-induced ion fluxes in dorsal root ganglion cells in culture. J Neurosci, 1988. 8(9): p. 3208-20.
- Cesare, P. and P. McNaughton, A novel heat-activated current in nociceptive neurons and its sensitization by bradykinin. Proc Natl Acad Sci U S A, 1996. 93(26): p. 15435-9.
- Reichling, D.B. and J.D. Levine, Heat transduction in rat sensory neurons by calcium- dependent activation of a cation channel. Proc Natl Acad Sci U S A, 1997. 94(13): p. 7006- 11.
- Caterina, M.J., M.A. Schumacher, M. Tominaga, T.A. Rosen, J.D. Levine, and D. Julius, The capsaicin receptor: a heat-activated ion channel in the pain pathway. Nature, 1997. 389(6653): p. 816-24.
- Clapham, D.E., TRP channels as cellular sensors. Nature, 2003. 426(6966): p. 517-24.
- Voets, T., K. Talavera, G. Owsianik, and B. Nilius, Sensing with TRP channels. Nat Chem Biol, 2005. 1(2): p. 85-92.
- Tominaga, M., M.J. Caterina, A.B. Malmberg, T.A. Rosen, H. Gilbert, K. Skinner, B.E. Raumann, A.I. Basbaum, and D. Julius, The cloned capsaicin receptor integrates multiple pain-producing stimuli. Neuron, 1998. 21(3): p. 531-43.
- Caterina, M.J., A. Leffler, A.B. Malmberg, W.J. Martin, J. Trafton, K.R. Petersen-Zeitz, M. Koltzenburg, A.I. Basbaum, and D. Julius, Impaired nociception and pain sensation in mice lacking the capsaicin receptor. Science, 2000. 288(5464): p. 306-13.
- Davis, J.B., J. Gray, M.J. Gunthorpe, J.P. Hatcher, P.T. Davey, P. Overend, M.H. Harries, J. Latcham, C. Clapham, K. Atkinson, S.A. Hughes, K. Rance, E. Grau, A.J. Harper, P.L. Pugh, D.C. Rogers, S. Bingham, A. Randall, and S.A. Sheardown, Vanilloid receptor-1 is essential for inflammatory thermal hyperalgesia. Nature, 2000. 405(6783): p. 183-187.
- Fernández-Carvajal, A., R. González-Muñiz, G. Fernández-Ballester, and A. Ferrer-Montiel, Investigational drugs in early phase clinical trials targeting thermotransient receptor potential (thermoTRP) channels. Expert Opin Investig Drugs, 2020. 29(11): p. 1209-1222.
- Manitpisitkul, P., A. Mayorga, K. Shalayda, M. De Meulder, G. Romano, C. Jun, and J.A. Moyer, Safety, Tolerability and Pharmacokinetic and Pharmacodynamic Learnings from a Double-Blind, Randomized, Placebo-Controlled, Sequential Group First-in-Human Study of the TRPV1 Antagonist, JNJ-38893777, in Healthy Men. Clinical Drug Investigation, 2015. 35(6): p. 353-363.
- Catterall, W.A., G. Wisedchaisri, and N. Zheng, The chemical basis for electrical signaling. Nat Chem Biol, 2017. 13(5): p. 455-463.
- Long, S.B., X. Tao, E.B. Campbell, and R. MacKinnon, Atomic structure of a voltage- dependent K+ channel in a lipid membrane-like environment. Nature, 2007. 450(7168): p. 376-82.
- Cao, E., M. Liao, Y. Cheng, and D. Julius, TRPV1 structures in distinct conformations reveal activation mechanisms. Nature, 2013. 504(7478): p. 113-8.
- Liao, M., E. Cao, D. Julius, and Y. Cheng, Structure of the TRPV1 ion channel determined by electron cryo-microscopy. Nature, 2013. 504(7478): p. 107-12.
- Kwon, D.H., F. Zhang, Y. Suo, J. Bouvette, M.J. Borgnia, and S.Y. Lee, Heat-dependent opening of TRPV1 in the presence of capsaicin. Nat Struct Mol Biol, 2021. 28(7): p. 554- 563.
- Hoffmann, T., K. Kistner, F. Miermeister, R. Winkelmann, J. Wittmann, M.J. Fischer, C. Weidner, and P.W. Reeh, TRPA1 and TRPV1 are differentially involved in heat nociception of mice. Eur J Pain, 2013. 17(10): p. 1472-82.
- Woodbury, C.J., M. Zwick, S. Wang, J.J. Lawson, M.J. Caterina, M. Koltzenburg, K.M. Albers, H.R. Koerber, and B.M. Davis, Nociceptors lacking TRPV1 and TRPV2 have normal heat responses. J Neurosci, 2004. 24(28): p. 6410-5.
- Vriens, J., G. Owsianik, T. Hofmann, S.E. Philipp, J. Stab, X. Chen, M. Benoit, F. Xue, A. Janssens, S. Kerselaers, J. Oberwinkler, R. Vennekens, T. Gudermann, B. Nilius, and T. Voets, TRPM3 is a nociceptor channel involved in the detection of noxious heat. Neuron, 2011. 70(3): p. 482-94.
- Bandell, M., G.M. Story, S.W. Hwang, V. Viswanath, S.R. Eid, M.J. Petrus, T.J. Earley, and Patapoutian, Noxious cold ion channel TRPA1 is activated by pungent compounds and bradykinin. Neuron, 2004. 41(6): p. 849-57.
- Jordt, S.E., D.M. Bautista, H.H. Chuang, D.D. McKemy, P.M. Zygmunt, E.D. Högestätt, I.D. Meng, and D. Julius, Mustard oils and cannabinoids excite sensory nerve fibres through the TRP channel ANKTM1. Nature, 2004. 427(6971): p. 260-5.
- Bautista, D.M., P. Movahed, A. Hinman, H.E. Axelsson, O. Sterner, E.D. Högestätt, D. Julius, S.E. Jordt, and P.M. Zygmunt, Pungent products from garlic activate the sensory ion channel TRPA1. Proc Natl Acad Sci U S A, 2005. 102(34): p. 12248-52.
- Kwan, K.Y., A.J. Allchorne, M.A. Vollrath, A.P. Christensen, D.S. Zhang, C.J. Woolf, and D.P. Corey, TRPA1 contributes to cold, mechanical, and chemical nociception but is not essential for hair-cell transduction. Neuron, 2006. 50(2): p. 277-89.
- Macpherson, L.J., B.H. Geierstanger, V. Viswanath, M. Bandell, S.R. Eid, S. Hwang, and A. Patapoutian, The pungency of garlic: activation of TRPA1 and TRPV1 in response to allicin. Curr Biol, 2005. 15(10): p. 929-34.
- Talavera, K., J.B. Startek, J. Alvarez-Collazo, B. Boonen, Y.A. Alpizar, A. Sanchez, R. Naert, and B. Nilius, Mammalian Transient Receptor Potential TRPA1 Channels: From Structure to Disease. Physiol Rev, 2020. 100(2): p. 725-803.
- Laursen, W.J., E.O. Anderson, L.J. Hoffstaetter, S.N. Bagriantsev, and E.O. Gracheva, Species-specific temperature sensitivity of TRPA1. Temperature (Austin, Tex.), 2015. 2(2): p. 214-226.
- Bautista, D.M., S.-E. Jordt, T. Nikai, P.R. Tsuruda, A.J. Read, J. Poblete, E.N. Yamoah, A.I. Basbaum, and D. Julius, TRPA1 Mediates the Inflammatory Actions of Environmental Irritants and Proalgesic Agents. Cell, 2006. 124(6): p. 1269-1282.
- Karashima, Y., K. Talavera, W. Everaerts, A. Janssens, K.Y. Kwan, R. Vennekens, B. Nilius, and T. Voets, TRPA1 acts as a cold sensor in vitro and in vivo. Proc Natl Acad Sci U S A, 2009. 106(4): p. 1273-8.
- Moparthi, L., T.I. Kichko, M. Eberhardt, E.D. Högestätt, P. Kjellbom, U. Johanson, P.W. Reeh, A. Leffler, M.R. Filipovic, and P.M. Zygmunt, Human TRPA1 is a heat sensor displaying intrinsic U-shaped thermosensitivity. Sci Rep, 2016. 6: p. 28763.
- Yarmolinsky, D.A., Y. Peng, L.A. Pogorzala, M. Rutlin, M.A. Hoon, and C.S. Zuker, Coding and Plasticity in the Mammalian Thermosensory System. Neuron, 2016. 92(5): p. 1079- 1092.
- Vandewauw, I., K. De Clercq, M. Mulier, K. Held, S. Pinto, N. Van Ranst, A. Segal, T. Voet, R. Vennekens, K. Zimmermann, J. Vriens, and T. Voets, A TRP channel trio mediates acute noxious heat sensing. Nature, 2018. 555(7698): p. 662-666.
- Frenzel, H., J. Bohlender, K. Pinsker, B. Wohlleben, J. Tank, S.G. Lechner, D. Schiska, T. Jaijo, F. Rüschendorf, K. Saar, J. Jordan, J.M. Millán, M. Gross, and G.R. Lewin, A genetic basis for mechanosensory traits in humans. PLoS Biol, 2012. 10(5): p. e1001318.
- Johnson, K.O., I. Darian-Smith, and C. LaMotte, Peripheral neural determinants of temperature discrimination in man: a correlative study of responses to cooling skin. J Neurophysiol, 1973. 36(2): p. 347-70.
- McKemy, D.D., W.M. Neuhausser, and D. Julius, Identification of a cold receptor reveals a general role for TRP channels in thermosensation. Nature, 2002. 416(6876): p. 52-8.
- Peier, A.M., A. Moqrich, A.C. Hergarden, A.J. Reeve, D.A. Andersson, G.M. Story, T.J. Earley, I. Dragoni, P. McIntyre, S. Bevan, and A. Patapoutian, A TRP channel that senses cold stimuli and menthol. Cell, 2002. 108(5): p. 705-15.
- Bautista, D.M., J. Siemens, J.M. Glazer, P.R. Tsuruda, A.I. Basbaum, C.L. Stucky, S.E. Jordt, and D. Julius, The menthol receptor TRPM8 is the principal detector of environmental cold. Nature, 2007. 448(7150): p. 204-8.
- Colburn, R.W., M.L. Lubin, D.J. Stone, Y. Wang, D. Lawrence, Michael R. D’Andrea, M.R. Brandt, Y. Liu, C.M. Flores, and N. Qin, Attenuated Cold Sensitivity in TRPM8 Null Mice. Neuron, 2007. 54(3): p. 379-386.
- Dhaka, A., A.N. Murray, J. Mathur, T.J. Earley, M.J. Petrus, and A. Patapoutian, TRPM8 is required for cold sensation in mice. Neuron, 2007. 54(3): p. 371-8.
- Stevens, J.C. and K.K. Choo, Temperature sensitivity of the body surface over the life span. Somatosens Mot Res, 1998. 15(1): p. 13-28.
- Tan, C.H. and P.A. McNaughton, The TRPM2 ion channel is required for sensitivity to warmth. Nature, 2016. 536(7617): p. 460-3.
- Paricio-Montesinos, R., F. Schwaller, A. Udhayachandran, F. Rau, J. Walcher, R. Evangelista, J. Vriens, T. Voets, J.F.A. Poulet, and G.R. Lewin, The Sensory Coding of Warm Perception. Neuron, 2020. 106(5): p. 830-841.e3.
- Corey, D.P. and A.J. Hudspeth, Response latency of vertebrate hair cells. Biophysical journal, 1979. 26(3): p. 499-506.
- Delcour, A.H., B. Martinac, J. Adler, and C. Kung, Modified reconstitution method used in patch-clamp studies of Escherichia coli ion channels. Biophys J, 1989. 56(3): p. 631-6.
- Sukharev, S.I., P. Blount, B. Martinac, F.R. Blattner, and C. Kung, A large-conductance mechanosensitive channel in E. coli encoded by mscL alone. Nature, 1994. 368(6468): p. 265-8.
- Levina, N., S. Tötemeyer, N.R. Stokes, P. Louis, M.A. Jones, and I.R. Booth, Protection of Escherichia coli cells against extreme turgor by activation of MscS and MscL mechanosensitive channels: identification of genes required for MscS activity. Embo j, 1999. 18(7): p. 1730-7.
- McCarter, G.C., D.B. Reichling, and J.D. Levine, Mechanical transduction by rat dorsal root ganglion neurons in vitro. Neurosci Lett, 1999. 273(3): p. 179-82.
- Chalfie, M., Neurosensory mechanotransduction. Nature Reviews Molecular Cell Biology, 2009. 10(1): p. 44-52.
- Jin, P., L.Y. Jan, and Y.-N. Jan, Mechanosensitive Ion Channels: Structural Features Relevant to Mechanotransduction Mechanisms. Annual Review of Neuroscience, 2020. 43(1): p. 207-229.
- Kefauver, J.M., A.B. Ward, and A. Patapoutian, Discoveries in structure and physiology of mechanically activated ion channels. Nature, 2020. 587(7835): p. 567-576.
- Ranade, S.S., R. Syeda, and A. Patapoutian, Mechanically Activated Ion Channels. Neuron, 2015. 87(6): p. 1162-1179.
- Coste, B., J. Mathur, M. Schmidt, T.J. Earley, S. Ranade, M.J. Petrus, A.E. Dubin, and A. Patapoutian, Piezo1 and Piezo2 are essential components of distinct mechanically activated cation channels. Science, 2010. 330(6000): p. 55-60.
- Ikeda, R., M. Cha, J. Ling, Z. Jia, D. Coyle, and J.G. Gu, Merkel cells transduce and encode tactile stimuli to drive Aβ-afferent impulses. Cell, 2014. 157(3): p. 664-75.
- Maksimovic, S., M. Nakatani, Y. Baba, A.M. Nelson, K.L. Marshall, S.A. Wellnitz, P. Firozi, S.H. Woo, S. Ranade, A. Patapoutian, and E.A. Lumpkin, Epidermal Merkel cells are mechanosensory cells that tune mammalian touch receptors. Nature, 2014. 509(7502): p. 617-21.
- Woo, S.H., S. Ranade, A.D. Weyer, A.E. Dubin, Y. Baba, Z. Qiu, M. Petrus, T. Miyamoto, K. Reddy, E.A. Lumpkin, C.L. Stucky, and A. Patapoutian, Piezo2 is required for Merkel-cell mechanotransduction. Nature, 2014. 509(7502): p. 622-6.
- Yamashita, Y. and H. Ogawa, Slowly adapting cutaneous mechanoreceptor afferent units associated with Merkel cells in frogs and effects of direct currents. Somatosens Mot Res, 1991. 8(1): p. 87-95.
- Ranade, S.S., S.H. Woo, A.E. Dubin, R.A. Moshourab, C. Wetzel, M. Petrus, J. Mathur, V. Bégay, B. Coste, J. Mainquist, A.J. Wilson, A.G. Francisco, K. Reddy, Z. Qiu, J.N. Wood, G.R. Lewin, and A. Patapoutian, Piezo2 is the major transducer of mechanical forces for touch sensation in mice. Nature, 2014. 516(7529): p. 121-5.
- Chesler, A.T., M. Szczot, D. Bharucha-Goebel, M. Čeko, S. Donkervoort, C. Laubacher, L.H. Hayes, K. Alter, C. Zampieri, C. Stanley, A.M. Innes, J.K. Mah, C.M. Grosmann, N. Bradley, D. Nguyen, A.R. Foley, C.E. Le Pichon, and C.G. Bönnemann, The Role of PIEZO2 in Human Mechanosensation. N Engl J Med, 2016. 375(14): p. 1355-1364.
- Haliloglu, G., K. Becker, C. Temucin, B. Talim, N. Küçükşahin, M. Pergande, S. Motameny, P. Nürnberg, U. Aydingoz, H. Topaloglu, and S. Cirak, Recessive PIEZO2 stop mutation causes distal arthrogryposis with distal muscle weakness, scoliosis and proprioception defects. J Hum Genet, 2017. 62(4): p. 497-501.
- Mahmud, A.A., N.A. Nahid, C. Nassif, M.S. Sayeed, M.U. Ahmed, M. Parveen, M.I. Khalil, M.M. Islam, Z. Nahar, F. Rypens, F.F. Hamdan, G.A. Rouleau, A. Hasnat, and J.L. Michaud, Loss of the proprioception and touch sensation channel PIEZO2 in siblings with a progressive form of contractures. Clin Genet, 2017. 91(3): p. 470-475.
- von Buchholtz, L.J., N. Ghitani, R.M. Lam, J.A. Licholai, A.T. Chesler, and N.J.P. Ryba, Decoding Cellular Mechanisms for Mechanosensory Discrimination. Neuron, 2021. 109(2): p. 285-298.e5.
- Woo, S.H., V. Lukacs, J.C. de Nooij, D. Zaytseva, C.R. Criddle, A. Francisco, T.M. Jessell, K.A. Wilkinson, and A. Patapoutian, Piezo2 is the principal mechanotransduction channel for proprioception. Nat Neurosci, 2015. 18(12): p. 1756-62.
- Ge, J., W. Li, Q. Zhao, N. Li, M. Chen, P. Zhi, R. Li, N. Gao, B. Xiao, and M. Yang, Architecture of the mammalian mechanosensitive Piezo1 channel. Nature, 2015. 527(7576): p. 64-9.
- Saotome, K., S.E. Murthy, J.M. Kefauver, T. Whitwam, A. Patapoutian, and A.B. Ward, Structure of the mechanically activated ion channel Piezo1. Nature, 2018. 554(7693): p. 481-486.
- Wang, L., H. Zhou, M. Zhang, W. Liu, T. Deng, Q. Zhao, Y. Li, J. Lei, X. Li, and B. Xiao, Structure and mechanogating of the mammalian tactile channel PIEZO2. Nature, 2019. 573(7773): p. 225-229.
- Zhao, Q., H. Zhou, S. Chi, Y. Wang, J. Wang, J. Geng, K. Wu, W. Liu, T. Zhang, M.Q. Dong, J. Wang, X. Li, and B. Xiao, Structure and mechanogating mechanism of the Piezo1 channel. Nature, 2018. 554(7693): p. 487-492.
- Guo, Y.R. and R. MacKinnon, Structure-based membrane dome mechanism for Piezo mechanosensitivity. Elife, 2017. 6.
- Jiang, Y., X. Yang, J. Jiang, and B. Xiao, Structural Designs and Mechanogating Mechanisms of the Mechanosensitive Piezo Channels. Trends Biochem Sci, 2021. 46(6): p. 472-488.
- Nonomura, K., S.H. Woo, R.B. Chang, A. Gillich, Z. Qiu, A.G. Francisco, S.S. Ranade, S.D. Liberles, and A. Patapoutian, Piezo2 senses airway stretch and mediates lung inflation- induced apnoea. Nature, 2017. 541(7636): p. 176-181.
- Min, S., R.B. Chang, S.L. Prescott, B. Beeler, N.R. Joshi, D.E. Strochlic, and S.D. Liberles, Arterial Baroreceptors Sense Blood Pressure through Decorated Aortic Claws. Cell Rep, 2019. 29(8): p. 2192-2201.e3.
- Zeng, W.Z., K.L. Marshall, S. Min, I. Daou, M.W. Chapleau, F.M. Abboud, S.D. Liberles, and Patapoutian, PIEZOs mediate neuronal sensing of blood pressure and the baroreceptor reflex. Science, 2018. 362(6413): p. 464-467.
- Alcaino, C., K.R. Knutson, A.J. Treichel, G. Yildiz, P.R. Strege, D.R. Linden, J.H. Li, A.B. Leiter, J.H. Szurszewski, G. Farrugia, and A. Beyder, A population of gut epithelial enterochromaffin cells is mechanosensitive and requires Piezo2 to convert force into serotonin release. Proc Natl Acad Sci U S A, 2018. 115(32): p. E7632-e7641.
- Wang, F., K. Knutson, C. Alcaino, D.R. Linden, S.J. Gibbons, P. Kashyap, M. Grover, R. Oeckler, P.A. Gottlieb, H.J. Li, A.B. Leiter, G. Farrugia, and A. Beyder, Mechanosensitive ion channel Piezo2 is important for enterochromaffin cell response to mechanical forces. J Physiol, 2017. 595(1): p. 79-91.
- Marshall, K.L., D. Saade, N. Ghitani, A.M. Coombs, M. Szczot, J. Keller, T. Ogata, I. Daou, L.T. Stowers, C.G. Bönnemann, A.T. Chesler, and A. Patapoutian, PIEZO2 in sensory neurons and urothelial cells coordinates urination. Nature, 2020. 588(7837): p. 290-295.
- Li, J., B. Hou, S. Tumova, K. Muraki, A. Bruns, M.J. Ludlow, A. Sedo, A.J. Hyman, L. McKeown, R.S. Young, N.Y. Yuldasheva, Y. Majeed, L.A. Wilson, B. Rode, M.A. Bailey, H.R. Kim, Z. Fu, D.A. Carter, J. Bilton, H. Imrie, P. Ajuh, T.N. Dear, R.M. Cubbon, M.T. Kearney, R.K. Prasad, P.C. Evans, J.F. Ainscough, and D.J. Beech, Piezo1 integration of vascular architecture with physiological force. Nature, 2014. 515(7526): p. 279-282.
- Ranade, S.S., Z. Qiu, S.H. Woo, S.S. Hur, S.E. Murthy, S.M. Cahalan, J. Xu, J. Mathur, M. Bandell, B. Coste, Y.S. Li, S. Chien, and A. Patapoutian, Piezo1, a mechanically activated ion channel, is required for vascular development in mice. Proc Natl Acad Sci U S A, 2014. 111(28): p. 10347-52.
- Retailleau, K., F. Duprat, M. Arhatte, S.S. Ranade, R. Peyronnet, J.R. Martins, M. Jodar, C. Moro, S. Offermanns, Y. Feng, S. Demolombe, A. Patel, and E. Honoré, Piezo1 in Smooth Muscle Cells Is Involved in Hypertension-Dependent Arterial Remodeling. Cell Rep, 2015. 13(6): p. 1161-1171.
- Rode, B., J. Shi, N. Endesh, M.J. Drinkhill, P.J. Webster, S.J. Lotteau, M.A. Bailey, N.Y. Yuldasheva, M.J. Ludlow, R.M. Cubbon, J. Li, T.S. Futers, L. Morley, H.J. Gaunt, K. Marszalek, H. Viswambharan, K. Cuthbertson, P.D. Baxter, R. Foster, P. Sukumar, A. Weightman, S.C. Calaghan, S.B. Wheatcroft, M.T. Kearney, and D.J. Beech, Piezo1 channels sense whole body physical activity to reset cardiovascular homeostasis and enhance performance. Nat Commun, 2017. 8(1): p. 350.
- Wang, S., R. Chennupati, H. Kaur, A. Iring, N. Wettschureck, and S. Offermanns, Endothelial cation channel PIEZO1 controls blood pressure by mediating flow-induced ATP release. J Clin Invest, 2016. 126(12): p. 4527-4536.
- Cahalan, S.M., V. Lukacs, S.S. Ranade, S. Chien, M. Bandell, and A. Patapoutian, Piezo1 links mechanical forces to red blood cell volume. Elife, 2015. 4.
- Li, X., L. Han, I. Nookaew, E. Mannen, M.J. Silva, M. Almeida, and J. Xiong, Stimulation of Piezo1 by mechanical signals promotes bone anabolism. Elife, 2019. 8.
- Sun, W., S. Chi, Y. Li, S. Ling, Y. Tan, Y. Xu, F. Jiang, J. Li, C. Liu, G. Zhong, D. Cao, X. Jin, D. Zhao, X. Gao, Z. Liu, B. Xiao, and Y. Li, The mechanosensitive Piezo1 channel is required for bone formation. Elife, 2019. 8.
- Wang, L., X. You, S. Lotinun, L. Zhang, N. Wu, and W. Zou, Mechanical sensing protein PIEZO1 regulates bone homeostasis via osteoblast-osteoclast crosstalk. Nat Commun, 2020. 11(1): p. 282.
- Kremeyer, B., F. Lopera, J.J. Cox, A. Momin, F. Rugiero, S. Marsh, C.G. Woods, N.G. Jones, K.J. Paterson, F.R. Fricker, A. Villegas, N. Acosta, N.G. Pineda-Trujillo, J.D. Ramírez, J. Zea, M.W. Burley, G. Bedoya, D.L. Bennett, J.N. Wood, and A. Ruiz-Linares, A gain-of-function mutation in TRPA1 causes familial episodic pain syndrome. Neuron, 2010. 66(5): p. 671- 80.
- Binder, A., D. May, R. Baron, C. Maier, T.R. Tölle, R.D. Treede, A. Berthele, F. Faltraco, H. Flor, J. Gierthmühlen, S. Haenisch, V. Huge, W. Magerl, C. Maihöfner, H. Richter, R. Rolke, Scherens, N. Uçeyler, M. Ufer, G. Wasner, J. Zhu, and I. Cascorbi, Transient receptor potential channel polymorphisms are associated with the somatosensory function in neuropathic pain patients. PLoS One, 2011. 6(3): p. e17387.
- Delle Vedove, A., M. Storbeck, R. Heller, I. Hölker, M. Hebbar, A. Shukla, O. Magnusson, S. Cirak, K.M. Girisha, M. O’Driscoll, B. Loeys, and B. Wirth, Biallelic Loss of Proprioception- Related PIEZO2 Causes Muscular Atrophy with Perinatal Respiratory Distress, Arthrogryposis, and Scoliosis. Am J Hum Genet, 2016. 99(5): p. 1206-1216.
- Szczot, M., J. Liljencrantz, N. Ghitani, A. Barik, R. Lam, J.H. Thompson, D. Bharucha-Goebel, D. Saade, A. Necaise, S. Donkervoort, A.R. Foley, T. Gordon, L. Case, M.C. Bushnell, C.G. Bönnemann, and A.T. Chesler, PIEZO2 mediates injury-induced tactile pain in mice and humans. Sci Transl Med, 2018. 10(462).
- Case, L.K., J. Liljencrantz, N. Madian, A. Necaise, J. Tubbs, M. McCall, M.L. Bradson, M. Szczot, M.H. Pitcher, N. Ghitani, E. Frangos, J. Cole, D. Bharucha-Goebel, D. Saade, T. Ogata, S. Donkervoort, A.R. Foley, C.G. Bönnemann, H. Olausson, M.C. Bushnell, and A.T. Chesler, Innocuous pressure sensation requires A-type afferents but not functional ΡΙΕΖΟ2 channels in humans. Nat Commun, 2021. 12(1): p. 657.
- Nagi, S.S., A.G. Marshall, A. Makdani, E. Jarocka, J. Liljencrantz, M. Ridderström, S. Shaikh, F. O’Neill, D. Saade, S. Donkervoort, A.R. Foley, J. Minde, M. Trulsson, J. Cole, C.G. Bönnemann, A.T. Chesler, M.C. Bushnell, F. McGlone, and H. Olausson, An ultrafast system for signaling mechanical pain in human skin. Sci Adv, 2019. 5(7): p. eaaw1297.
- Coste, B., G. Houge, M.F. Murray, N. Stitziel, M. Bandell, M.A. Giovanni, A. Philippakis, A. Hoischen, G. Riemer, U. Steen, V.M. Steen, J. Mathur, J. Cox, M. Lebo, H. Rehm, S.T. Weiss, J.N. Wood, R.L. Maas, S.R. Sunyaev, and A. Patapoutian, Gain-of-function mutations in the mechanically activated ion channel PIEZO2 cause a subtype of Distal Arthrogryposis. Proc Natl Acad Sci U S A, 2013. 110(12): p. 4667-72.
- McMillin, M.J., A.E. Beck, J.X. Chong, K.M. Shively, K.J. Buckingham, H.I. Gildersleeve, M.I. Aracena, A.S. Aylsworth, P. Bitoun, J.C. Carey, C.L. Clericuzio, Y.J. Crow, C.J. Curry, K. Devriendt, D.B. Everman, A. Fryer, K. Gibson, M.L. Giovannucci Uzielli, J.M. Graham, Jr., J.G. Hall, J.T. Hecht, R.A. Heidenreich, J.A. Hurst, S. Irani, I.P. Krapels, J.G. Leroy, D. Mowat, G.T. Plant, S.P. Robertson, E.K. Schorry, R.H. Scott, L.H. Seaver, E. Sherr, M. Splitt, H. Stewart, C. Stumpel, S.G. Temel, D.D. Weaver, M. Whiteford, M.S. Williams, H.K. Tabor, J.D. Smith, J. Shendure, D.A. Nickerson, and M.J. Bamshad, Mutations in PIEZO2 cause Gordon syndrome, Marden-Walker syndrome, and distal arthrogryposis type 5. Am J Hum Genet, 2014. 94(5): p. 734-44.
- Alisch, F., A. Weichert, K. Kalache, V. Paradiso, A.C. Longardt, C. Dame, K. Hoffmann, and D. Horn, Familial Gordon syndrome associated with a PIEZO2 mutation. Am J Med Genet A, 2017. 173(1): p. 254-259.
- Fotiou, E., S. Martin-Almedina, M.A. Simpson, S. Lin, K. Gordon, G. Brice, G. Atton, I. Jeffery, D.C. Rees, C. Mignot, J. Vogt, T. Homfray, M.P. Snyder, S.G. Rockson, S. Jeffery, P.S. Mortimer, S. Mansour, and P. Ostergaard, Novel mutations in PIEZO1 cause an autosomal recessive generalized lymphatic dysplasia with non-immune hydrops fetalis. Nat Commun, 2015. 6: p. 8085.
- Lukacs, V., J. Mathur, R. Mao, P. Bayrak-Toydemir, M. Procter, S.M. Cahalan, H.J. Kim, M. Bandell, N. Longo, R.W. Day, D.A. Stevenson, A. Patapoutian, and B.L. Krock, Impaired PIEZO1 function in patients with a novel autosomal recessive congenital lymphatic dysplasia. Nat Commun, 2015. 6: p. 8329.
- Albuisson, J., S.E. Murthy, M. Bandell, B. Coste, H. Louis-Dit-Picard, J. Mathur, M. Fénéant- Thibault, G. Tertian, J.P. de Jaureguiberry, P.Y. Syfuss, S. Cahalan, L. Garçon, F. Toutain, P. Simon Rohrlich, J. Delaunay, V. Picard, X. Jeunemaitre, and A. Patapoutian, Dehydrated hereditary stomatocytosis linked to gain-of-function mutations in mechanically activated PIEZO1 ion channels. Nat Commun, 2013. 4: p. 1884.
- Andolfo, I., S.L. Alper, L. De Franceschi, C. Auriemma, R. Russo, L. De Falco, F. Vallefuoco, M.R. Esposito, D.H. Vandorpe, B.E. Shmukler, R. Narayan, D. Montanaro, M. D’Armiento, A. Vetro, I. Limongelli, O. Zuffardi, B.E. Glader, S.L. Schrier, C. Brugnara, G.W. Stewart, J. Delaunay, and A. Iolascon, Multiple clinical forms of dehydrated hereditary stomatocytosis arise from mutations in PIEZO1. Blood, 2013. 121(19): p. 3925-35, s1-12.
- Zarychanski, R., V.P. Schulz, B.L. Houston, Y. Maksimova, D.S. Houston, B. Smith, J. Rinehart, and P.G. Gallagher, Mutations in the mechanotransduction protein PIEZO1 are associated with hereditary xerocytosis. Blood, 2012. 120(9): p. 1908-15.
- Ma, S., S. Cahalan, G. LaMonte, N.D. Grubaugh, W. Zeng, S.E. Murthy, E. Paytas, R. Gamini, V. Lukacs, T. Whitwam, M. Loud, R. Lohia, L. Berry, S.M. Khan, C.J. Janse, M. Bandell, C. Schmedt, K. Wengelnik, A.I. Su, E. Honore, E.A. Winzeler, K.G. Andersen, and A. Patapoutian, Common PIEZO1 Allele in African Populations Causes RBC Dehydration and Attenuates Plasmodium Infection. Cell, 2018. 173(2): p. 443-455.e12.
- Nguetse, C.N., N. Purington, E.R. Ebel, B. Shakya, M. Tetard, P.G. Kremsner, T.P. Velavan, and E.S. Egan, A common polymorphism in the mechanosensitive ion channel PIEZO1 is associated with protection from severe malaria in humans. Proc Natl Acad Sci U S A, 2020. 117(16): p. 9074-9081.
- Ma, S., A.E. Dubin, Y. Zhang, S.A.R. Mousavi, Y. Wang, A.M. Coombs, M. Loud, I. Andolfo, and A. Patapoutian, A role of PIEZO1 in iron metabolism in mice and humans. Cell, 2021. 184(4): p. 969-982.e13.
Nobel Prizes and laureates
Six prizes were awarded for achievements that have conferred the greatest benefit to humankind. The 12 laureates' work and discoveries range from proteins' structures and machine learning to fighting for a world free of nuclear weapons.
See them all presented here.