Popular information
Popular science background:
Cosmic chirps (pdf)
Populärvetenskaplig information:
Kosmiskt kvitter (pdf)
The Nobel Prize in Physics 2017
On 14 September 2015, the LIGO detectors in the USA saw space vibrate with gravitational waves for the very first time. Although the signal was extremely weak when it reached Earth, it is already promising a revolution in astrophysics. Gravitational waves are an entirely new way of following the most violent events in space and testing the limits of our knowledge.
Cosmic chirps
The gravitational waves that have now been observed were created in a ferocious collision between two black holes, more than a thousand million years ago. Albert Einstein was right again. A century had passed since gravitational waves were predicted by his general theory of relativity, but he had always been doubtful whether they could ever be captured.
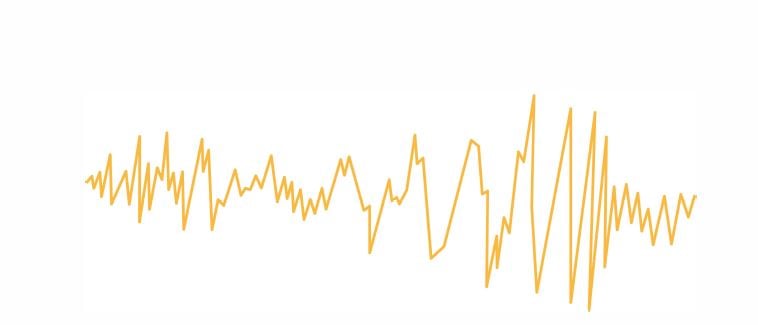
Figure 1. The frst gravitational wave ever detected.
LIGO, the Laser Interferometer Gravitational-Wave Observatory, is a collaborative project with over one thousand researchers from more than twenty countries. Together, they have realised a vision that is almost ffty years old. The 2017 Nobel Laureates have, with their enthusiasm and determination, each been invaluable to the success of LIGO. Pioneers Rainer Weiss and Kip S. Thorne, together with Barry C. Barish, the scientist and leader who brought the project to completion, have ensured that more than four decades of efort led to gravitational waves fnally being observed.
Rumours began to circulate around five months before the international research group had finished refining its calculations, but they did not dare to announce their findings until 11 February 2016. The LIGO researchers set several records with their very first discovery; besides the first ever observation of gravitational waves, the entire course of events was the first indication that space contains medium-sized black holes of between 30 and 60 solar masses and that they can merge. For a short moment, the gravitational radiation from the colliding black holes was many times stronger than the collected light of all the stars in the visible universe.
Spacetime vibrates
It was completely dark. But not completely still. Tremors from two black holes colliding shook all of spacetime. Like ripples from a pebble thrown into water, gravitational waves from the impact spread through the cosmos. It took time for them to reach us. Despite moving at the speed of light, the fastest possible, it took more than a thousand million years for these waves to arrive here on Earth. On 14 September 2015, at 11.51 CET, a gentle wobble in the light pattern at America’s twin LIGO laboratories revealed the drama that unfolded long ago and far away, 1.3 billion lightyears from Earth.
LIGO is no ordinary telescope for detecting light and other electromagnetic radiation from space. It is an instrument for listening to space’s gravitational waves; even if gravitational waves are tremors in spacetime itself, and not sound waves, their frequency is equivalent to those we can hear with our human ears.
For decades, physicists have tried to detect these gravitational waves that shake the universe, as Albert Einstein described them one hundred years ago. He explained that space and time are malleable, and that the combined four-dimensional spacetime vibrates with gravitational waves that are always created when a mass accelerates – like when an ice-skater pirouettes, a star explodes in a distant galaxy or a pair of black holes rotate around each other.
Like gravitational waves, black holes are also described by Einstein’s general theory of relativity from 1915. For more than fifty years, most researchers remained convinced that black holes only existed as solutions to Einstein’s equations, and were not actually out there in space. The theory of relativity explains gravity as a curvature of spacetime. Where gravity is extremely strong, the curvature can become so great that a black hole forms. Black holes are the most bizarre objects in spacetime – nothing can escape them, not even light. They are therefore a constant source of mystery in physics.
Gravitational waves bring the hope of observing something previously unthought-of, but it was long unclear whether the mysteries of spacetime would ever be solved. For many years, Albert Einstein was convinced it would never be possible to measure the gravitational waves and was unsure whether the waves were real or just a mathematical illusion. His contemporary colleague, Arthur Eddington, was even more sceptical and pointed out that gravitational waves appeared “to propagate at the speed of thought”.
The existence of gravitational waves became more accepted towards the end of the 1950s, when new calculations demonstrated that they actually carry energy and therefore should, in principle, be measurable. One piece of indirect evidence came in the 1970s, when the American astronomers Joseph Taylor and Russell Hulse used a large radio telescope to observe a pair of extremely dense stars, a double pulsar. They were able to show that the stars rotated around each other at increasing speed, while losing energy and moving closer together. The amount of lost energy corresponded to the theoretical calculations for gravitational waves. Joseph Taylor and Russell Hulse were awarded the Nobel Prize in Physics in 1993.
However, obtaining direct evidence of gravitational waves requires direct observations of the waves. But spacetime is rigid and not easy to shake up, so only the most violent cosmic processes can cause gravitational waves large enough to measure. Yet their amplitude is tiny: detecting them is like measuring the distance to a star ten lightyears away with a precision equivalent to the diameter of a strand of hair. Also, even if the entire universe is constantly vibrating with gravitational waves, the most explosive events rarely occur in our galaxy. We have to look further afield.
Gravitational waves reveal the past
Now it has happened – gravitational waves have been caught in LIGO’s trap. The two black holes that finally collided had moved in circles around each other ever since their creation, early in the history of the universe. With every cycle, they swept spacetime into a spiral, a spacetime disturbance which propagated further and further out into space in the form of gravitational waves.
The waves carried away energy, causing the black holes to move closer to one another. The closer their spiralling motion brought them, the faster the black holes rotated and the more energy was sent out in an accelerating dance that continued for many millions of years. At the very end, in a fraction of a second, the horizons of the black holes touched each other, and the holes swung towards their fateful end at almost the speed of light. As they merged, all the vibrations died away, leaving behind a single rotating black hole with no traces visible of its dramatic inception.
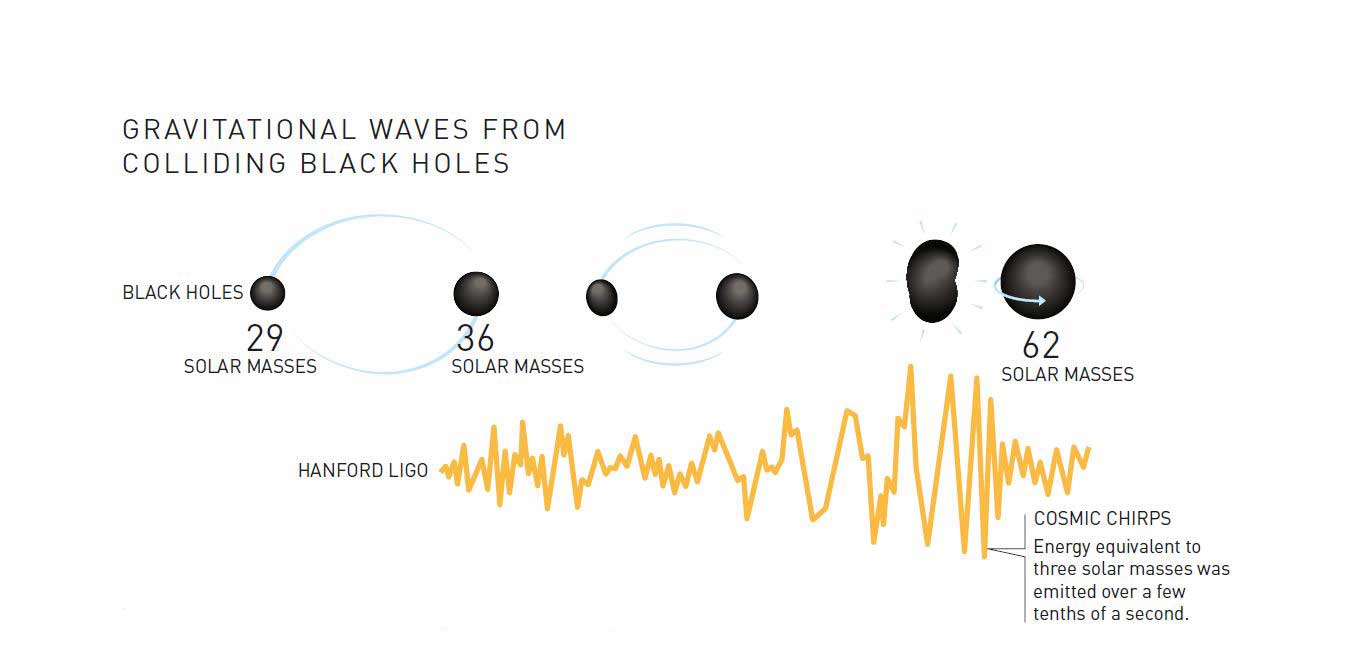
Figure 2. The two black holes emitted gravitational waves for many million years as they rotated around each other. They got closer and closer, before merging to become one black hole in a few tenths of a second. The waves then reached a crescendo which, to us on Earth, 1.3 billion lightyears away, sounded like cosmic chirps that came to an abrupt stop.
But the memory of this union is not completely lost – its history remains in ripples of spacetime. Gravitational waves, which rhythmically stretch and squeeze space, change tone as their message alters. If we could hear all the waves and not only the strongest ones, the entire universe would be full of music, like birds chirping in a forest, with a louder tone here and a quieter one there. After billions of years, as the duo of black holes accelerates towards their final chaotic collision, there is a crescendo before the tones fade away to a silence that reveals nothing.
Only the final few chirps can now be heard through space. Why are they so quiet? This is because their source was so very far away and gravitational waves, like light waves, weaken with distance. So when gravitational waves arrive here, their strength has significantly declined – the stretch in the fabric of spacetime that the LIGO detector had to catch as the wave passed Earth was thousands of times smaller than an atomic nucleus.
LIGO – a gigantic interferometer
The dream had been around for over fifty years, and the road to success was long, winding and sometimes tough for many of the researchers involved. One of the first detectors for capturing gravitational waves resembled a tuning fork, sensitive to waves of a particular frequency. But Joseph Weber at the University of Maryland in Washington could only guess the frequency at which the black holes would sing their swan song. He built the very first detector in the 1960s, but at that time many people doubted that gravitational waves and black holes even existed. So it was a sensation when, in the 1970s, Weber claimed to have heard these final tones. However, no one was able to repeat Weber’s results and his observations are considered to be false alarms.
In the mid-1970s, despite widespread scepticism, both Kip Thorne and Rainer Weiss were firmly convinced that gravitational waves could be detected and bring about a revolution in our knowledge of the universe. Rainer Weiss had already analysed possible sources of background noise that would disturb their measurements. He had also designed a detector, a laser-based interferometer, which would overcome this noise.
While Rainer Weiss was developing his detectors at MIT in Cambridge, outside Boston, Kip Thorne also started working with Ronald Drever, who built his first prototypes in Glasgow, Scotland. Drever eventually moved to join Thorne at Caltech in Los Angeles. Together, Weiss, Thorne and Drever formed a trio that pioneered development for many years. Drever ultimately ended up outside the project’s primary path, but he was able to experience its first discovery before passing away at home in Scotland in March 2017.
Instead of Weber’s tuning fork design, Weiss, Thorne and Drever developed another instrument, a laser-based interferometer. The principle has long been known: an interferometer consists of two arms that form an L. At the corner and the ends of the L, massive mirrors are suspended in a sophi ticated device. A passing gravitational wave affects the interferometer’s arms differently – when one arm is compressed, the other is stretched.
A laser beam that bounces between the mirrors measures the change in the lengths of the arms. If nothing happens, the bouncing light beams from the laser cancel each other out when they meet at the corner of the L. However, if either of the interferometer’s arms changes length, the light travels different distances, so the light waves lose synchronisation and the resulting light’s intensity changes where the beams meet.
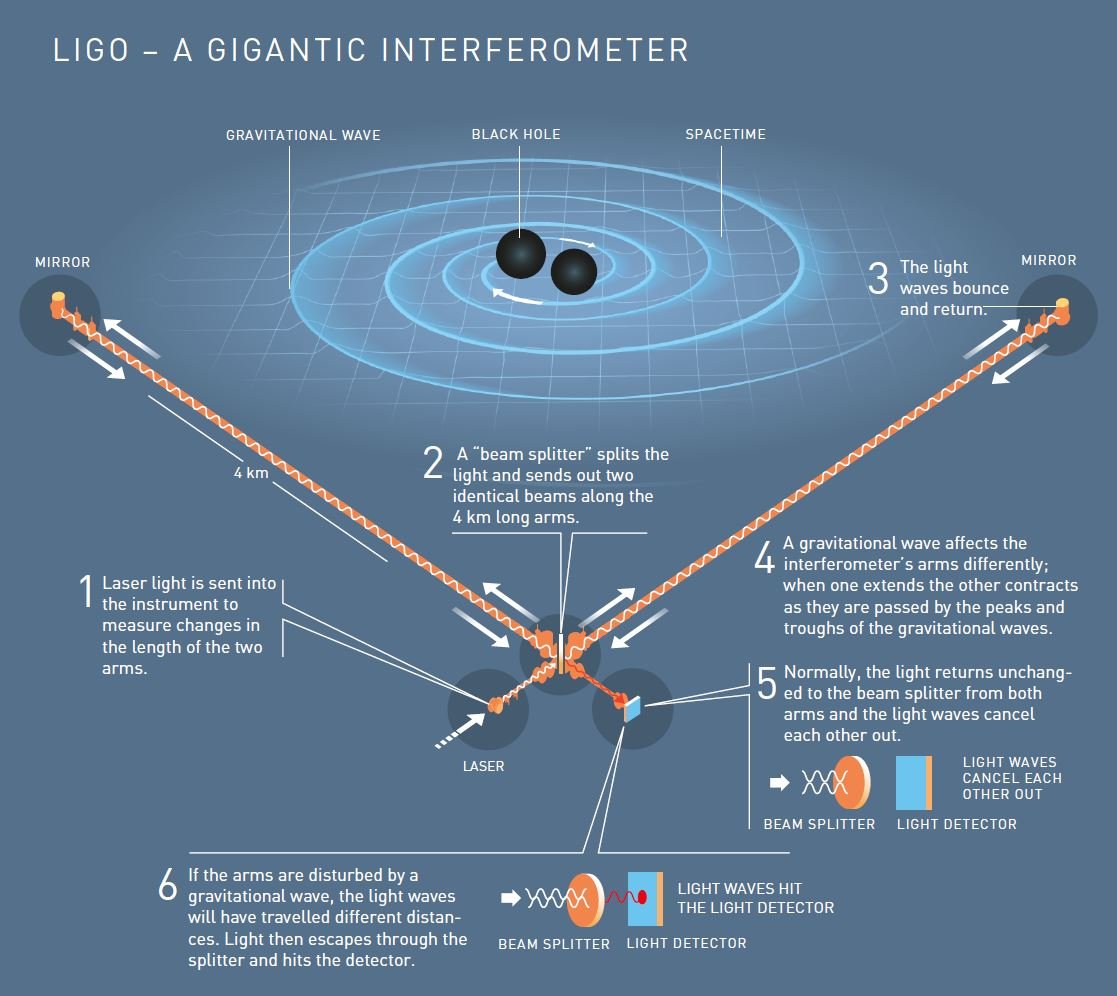
Figure 3. How to catch a gravitational wave. The world’s frst captured gravitational waves were created in a violent collision between two black holes, 1.3 billion lightyears away. When these waves passed the Earth, 1.3 billion years later, they had weakened considerably: the disturbance in spacetime that LIGO measured was thousands of times smaller than an atomic nucleus.
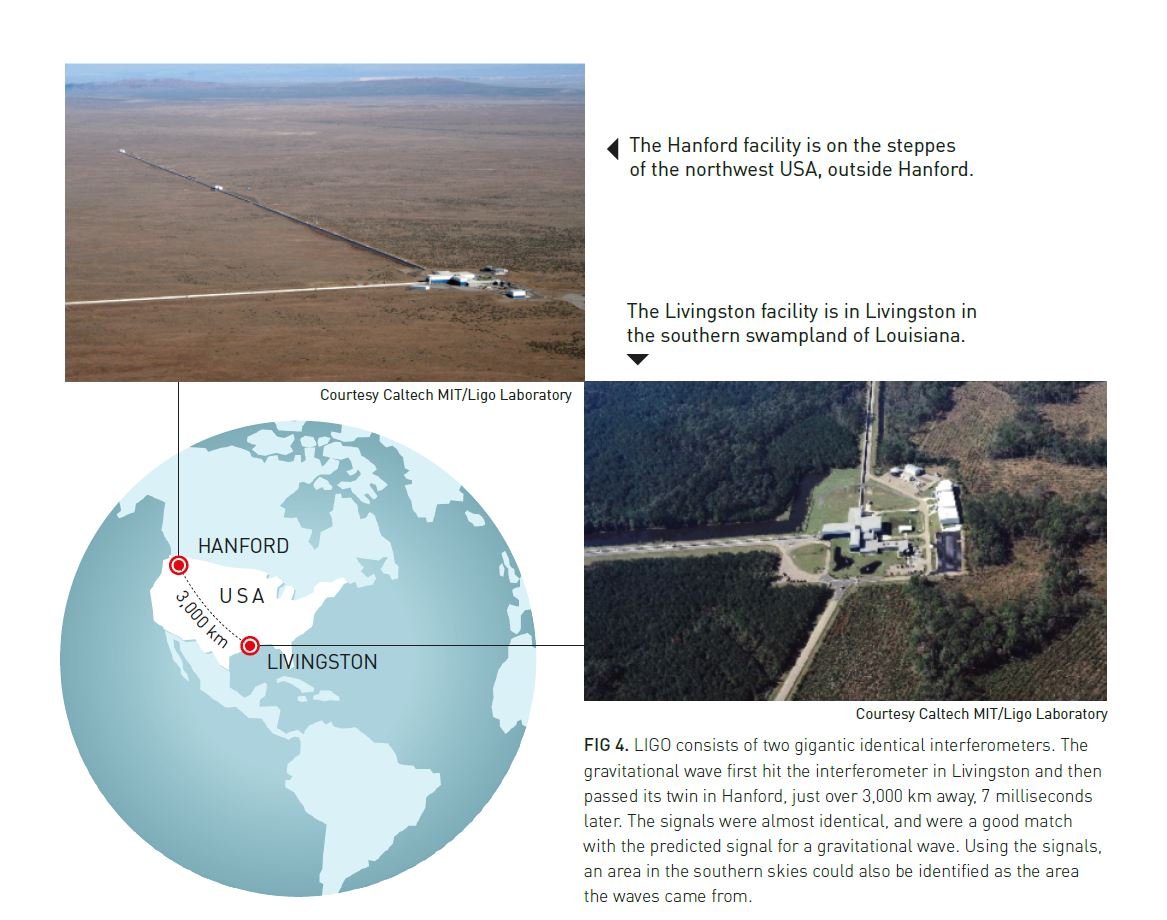
The idea was fairly simple, but the devil was in the details, so it took over forty years to realise. Large-scale instruments are required to measure microscopic changes of lengths less than an atom’s nucleus. The plan was to build two interferometers, each with four-kilometre-long arms along which the laser beam bounces many times, thus extending the path of the light and increasing the chance of detecting any tiny stretches in spacetime. LIGO is located in the steppes of the USA’s northwest, outside Hanford, Washington, with a twin facility three thousand kilometres to the south, in the swamps of Livingston, Louisiana.
It took years of developing the most sensitive instrument ever to be able to distinguish gravitational waves from all the background noise. This required sophisticated analysis and advanced theory, for which Kip Thorne was the expert. But the highest levels of creative engineering and craftsmanship are necessary to build ingenious instruments, and this was Rainer Weiss’ pioneering contribution. The laser light’s wavelength and intensity must be as stable as possible, and the beam must hit the suspended mirrors precisely. They should hardly shake at all, not even when leaves fall from nearby trees, a child runs by or a lorry passes on a distant road. At the same time, these hanging mirrors must be free to swing with the passage of gravitational waves. The thermal motion of atoms on the surface of the mirrors must be compensated for, as well as quantum effects in the laser. It was necessary to develop new laser technology and invent new materials, as well as construct gigantic vacuum tubes, seismic isolation and other vital technology far beyond what had previously been achieved.
Running such a project on a small scale was no longer possible and a new approach was needed. In 1994, when Barry Barish took over as leader for LIGO, he transformed the small research group of about 40 people into a large-scale international collaboration with more than a thousand participants. He searched for the necessary expertise and brought in numerous research groups from many countries. The impossible dream could only come true through the collaborative efforts of big science.
The signal arrived immediately
In September 2015, LIGO was about to start up again after an upgrade that had lasted several years. Now equipped with tenfold more powerful lasers, mirrors weighing 40 kilos, highly advanced noise filtering, and one of the world’s largest vacuum systems, it captured a wave signal a few days before the experiment was set to officially start. The wave first passed the Livingston facility and then, 7 milliseconds later – moving at the speed of light – it appeared at Hanford, three thousand kilometres away.
A message from the computerised system was sent early in the morning on 14 September 2015. Everyone in the USA was sleeping, but in Hannover in Germany it was 11:51 and Marco Drago, a young physicist at the Max Planck Institute for Gravitational Physics, was getting ready for lunch. The curves he glimpsed looked exactly like those he had practiced recognising so many times. Could he really be the first person in the world to see gravitational waves? Or was it just a false alarm, one of the occasional blind tests about which only a few people knew?
The wave’s form was exactly as predicted, and it was not a test. Everything fit perfectly. The pioneers, now in their 80s, and their LIGO colleagues were finally able to hear the music of their dreams, like a glissando, or a bird chirping its solitary song. It was almost too good to be true, but it was not until February the following year that they were allowed to reveal the news to anyone, even their families.
The well-kept secret called GW 150914 met all their expectations. From the signal, researchers could work out that the black holes were 29 and 36 times heavier than the Sun, but no larger than 200 kilometres in diameter. They merged to form a black hole of around 62 solar masses, so that during a few tenths of a second they were able to radiate energy in the form of gravitational waves equivalent to three solar masses. This made GW 150914 the most powerful radiant object in the universe for that short moment. The signal also indicates the area in the southern skies where the violent event occurred, 1.3 billion lightyears away. This means that the collision happened 1.3 billion years ago, at the time when life on Earth was taking the step from unicellular to multicellular organisms.
LIGO has observed another two similar events since the first discovery. The European sister facility, VIRGO, outside Pisa in Italy, joined LIGO in August 2017 and they announced their first collaborative discovery on 27 September. All three detectors observed the same cosmic gravitational waves on 14 August 2017; they came from two medium-sized black holes that collided 1.8 billion years ago.
The detectors have now seen the universe shake four times and many more discoveries are expected. India and Japan are also building new gravitational wave observatories. With several experiments located far apart, researchers should be able to precisely identify where the signals are coming from. Observations of gravitational waves can then be followed by studies using optical telescopes, X-ray telescopes, or other types of telescopes.
So far all sorts of electromagnetic radiation and particles, like cosmic rays or neutrinos, have given us knowledge about the universe. Gravitational waves, however, are direct testimony to disturbances in spacetime itself. This is something completely new and different, opening up unseen worlds. A wealth of discoveries awaits those who succeed in capturing gravitational waves and interpreting their message.
LINKS AND FURTHER READING
Additional information on this year’s prizes, including a scientific background in English, is available on the website of the Royal Swedish Academy of Sciences, www.kva.se, and at http://nobelprize.org. There you can watch video footage of the press conferences, the Nobel Lectures and more. Information on exhibitions and activities related to the Nobel Prizes and the Prize in Economic Sciences is available at www.nobelmuseum.se.
LIGO websites
www.ligo.org
www.ligo.caltech.edu
www.advancedligo.mit.edu
Books
Collins, H. (2017) Gravity’s Kiss: The Detection of Gravitational Waves, MIT Press.
Bartusiak, M. (2017) Einstein’s Unfinished Symphony: The Story of a Gamble, Two Black Holes, and a New Age of Astronomy, Yale University Press
Levin, J. (2016) Black Hole Blues and Other Songs from Outer Space. Knopf
Kennefick, D. (2007) Traveling at the Speed of Thought: Einstein and the Quest for Gravitational Waves. Princeton University Press
Collins, H. (2004) Gravity’s Shadow: The Search for Gravitational Waves. University of Chicago Press.
Thorne, K. S. (1994) Black Holes and Time Warps: Einstein’s Outrageous Legacy. W. W. Norton & Company
Videos
Hear the chirp/the sound of two black holes colliding
https://www.youtube.com/watch?time_continue=1&v=egfBaUdnAyQ
Barish, C. B. (2017, 13 January) The Long Odyssey from Einstein to Gravitational Waves. The Royal Swedish Academy of Sciences
http://kva.screen9.tv/media/1OtpBoqgCGvsg_ifTD8kkg/the-long-odyssey-from-einstein-to-gravitational-waves
Thorne, K. S. (2016, 25 May) Disturbed Kerr Black Holes. The Royal Swedish Academy of Sciences
http://kva.screen9.tv/media/wkdjHKIXoijrB-sc0i9LKA/disturbed-kerr-black-holes
LIGO Generations (2015) Kai Staats
https://vimeo.com/115282354
LIGO, a passion for understanding (2014) Kai Staats
https://vimeo.com/88437726
Scientific article
Abbott, B. P. et al (2016) Observation of Gravitational Waves from a Binary Black Hole Merger, Physical Review Letters, vol. 116, 061102
The Royal Swedish Academy of Sciences has decided to award the Nobel Prize in Physics 2017
with one half to
RAINER WEISS
Born 1932 in Berlin, Germany. Ph.D. 1962 from the Massachusetts Institute of Technology, MIT, Cambridge, MA, USA. Professor of Physics, Massachusetts Institute of Technology, MIT, Cambridge, MA, USA.
http://web.mit.edu/physics/people/ faculty/weiss_rainer.html
“for theoretical discoveries in physical cosmology”
and the other half jointly to
BARRY C. BARISH
Born 1936 in Omaha, NE, USA. Ph.D. 1962 from the University of California, Berkeley, CA, USA. Linde Professor of Physics, California Institute of Technology, Pasadena, CA, USA.
https://labcit.ligo.caltech.edu/~BCBAct
and
KIP S. THORNE
Born 1940 in Logan, UT, USA. Ph.D. 1965 from Princeton University, NJ, USA. Feynman Professor of Theoretical Physics, California Institute of Technology, Pasadena, CA, USA.
https://www.its.caltech.edu/~kip/index. html/
“for decisive contributions to the LIGO detector and the observation of gravitational waves”
Science Editors: Olga Botner, Ulf Danielsson and Gunnar Ingelman, the Nobel Committee for Physics
Text: Joanna Rose
Translation: Clare Barnes
Illustrations: © Johan Jarnestad/The Royal Swedish Academy of Sciences
Editor: Joanna Rose
© The Royal Swedish Academy of Sciences
Nobel Prizes and laureates
Six prizes were awarded for achievements that have conferred the greatest benefit to humankind. The 12 laureates' work and discoveries range from proteins' structures and machine learning to fighting for a world free of nuclear weapons.
See them all presented here.